‘Amazing’ Math Bridge Extended Beyond Fermat’s Last Theorem
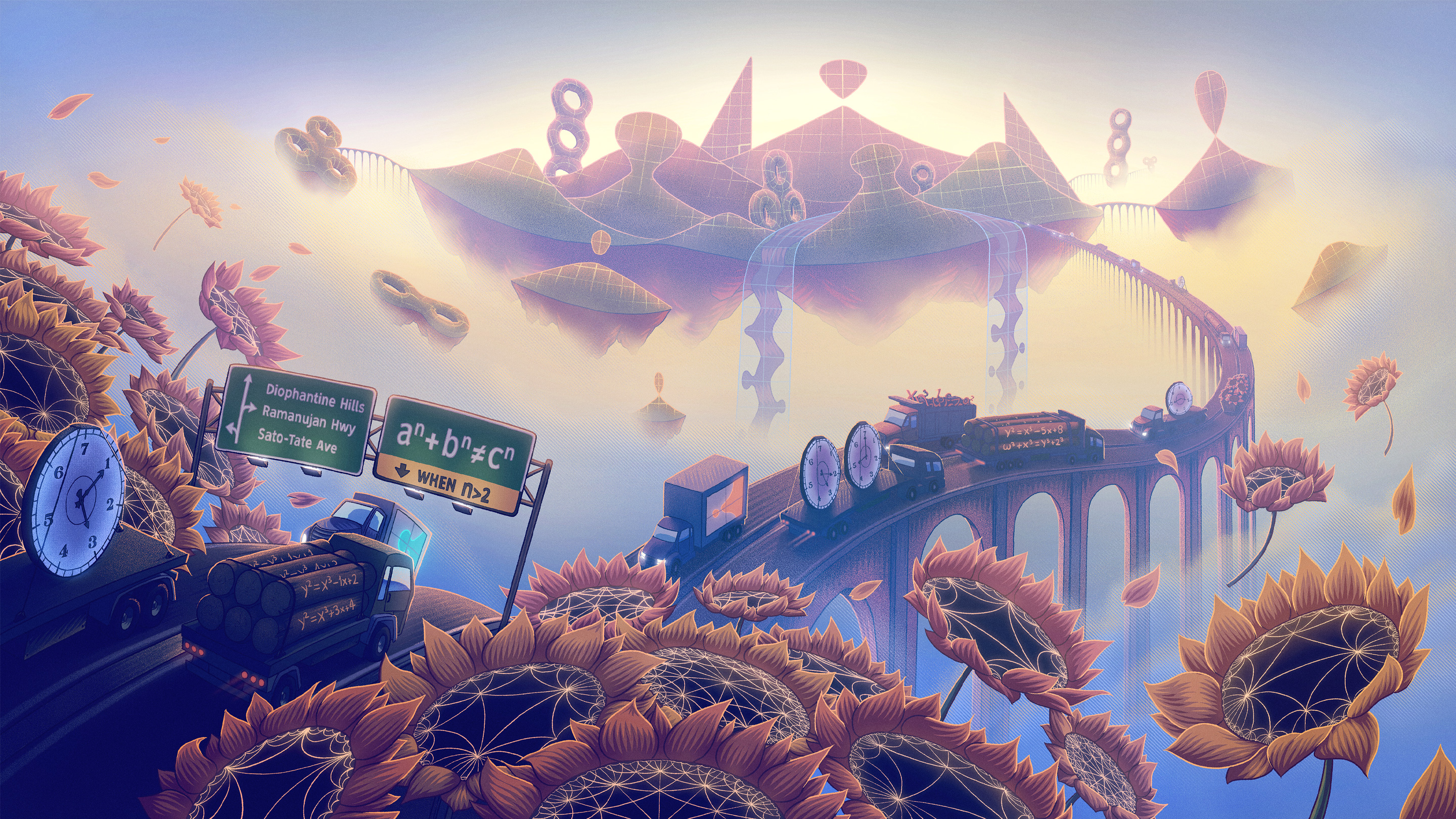
Daniel Castro Maia for Quanta Magazine
Introduction
When Andrew Wiles proved Fermat’s Last Theorem in the early 1990s, his proof was hailed as a monumental step forward not just for mathematicians but for all of humanity. The theorem is simplicity itself — it posits that xn + yn = zn has no positive whole-number solutions when n is greater than 2. Yet this simple claim tantalized legions of would-be provers for more than 350 years, ever since the French mathematician Pierre de Fermat jotted it down in 1637 in the margin of a copy of Diophantus’ Arithmetica. Fermat, notoriously, wrote that he had discovered “a truly marvelous proof, which this margin is too narrow to contain.” For centuries, professional mathematicians and amateur enthusiasts sought Fermat’s proof — or any proof at all.
The proof Wiles finally came up with (helped by Richard Taylor) was something Fermat would never have dreamed up. It tackled the theorem indirectly, by means of an enormous bridge that mathematicians had conjectured should exist between two distant continents, so to speak, in the mathematical world. Wiles’ proof of Fermat’s Last Theorem boiled down to establishing this bridge between just two little plots of land on the two continents. The proof, which was full of deep new ideas, set off a cascade of further results about the two sides of this bridge.
From this perspective, Wiles’ awe-inspiring proof solved just a minuscule piece of a much larger puzzle. His proof was “one of the best things in 20th-century mathematics,” said Toby Gee of Imperial College London. Yet “it was still only a tiny corner” of the conjectured bridge, known as the Langlands correspondence.
The full bridge would offer mathematicians the hope of illuminating vast swaths of mathematics by passing concepts back and forth across it. Many problems, including Fermat’s Last Theorem, seem difficult on one side of the bridge, only to transform into easier problems when shifted to the other side.
After Wiles came up with his proof, other mathematicians eagerly extended his bridge to slightly larger portions of the two continents. But then they hit a wall. There are two natural next directions for extending the bridge further, but for both, the Taylor-Wiles method faced what seemed like an insuperable barrier.
“People wanted to do this for a long time,” said Ana Caraiani of Imperial College London. But “we pretty much didn’t think it was possible.”
Now, two papers — representing the culmination of the efforts of more than a dozen mathematicians — have overcome this barrier, essentially solving both problems. Eventually, these findings may help mathematicians prove Fermat’s Last Theorem for some number systems beyond the positive whole numbers.
They are “pivotal results,” said Matthew Emerton of the University of Chicago. “There are some fundamental number-theoretic phenomena that are being revealed, and we’re just starting to understand what they are.”
Needle in a Vacuum
One side of the Langlands bridge focuses on some of the least complicated equations you can write down: “Diophantine” equations, which are combinations of variables, exponents and coefficients, such as y = x2 + 6x + 8, or x3 + y3 = z3. For millennia, mathematicians have tried to figure out which combinations of whole numbers satisfy a given Diophantine equation. They’re motivated primarily by how simple and natural this question is, although some of their work has recently had unforeseen applications in areas such as cryptography.
Since the time of the ancient Greeks, mathematicians have known how to find the whole-number solutions to Diophantine equations that have just two variables and no exponents higher than 2. But searching for whole-number solutions is anything but straightforward with equations that have larger exponents, starting with elliptic curves. These are equations that have y2 on the left and a combination of terms whose highest power is 3, like x3 + 4x + 7, on the right. They’re a “massively harder problem” than equations with lower exponents, Gee said.
On the other side of the bridge live objects called automorphic forms, which are akin to highly symmetric colorings of certain tilings. In the cases Wiles studied, the tiling might be something along the lines of M.C. Escher’s famous tessellations of a disk with fish or angels and devils that get smaller near the boundary. In the broader Langlands universe, the tiling might instead fill a three-dimensional ball or some other higher-dimensional space.
These two types of mathematical objects have completely different flavors. Yet in the middle of the 20th century, mathematicians started uncovering deep relationships between them, and by the early 1970s, Robert Langlands of the Institute for Advanced Study had conjectured that Diophantine equations and automorphic forms match up in a very specific manner.
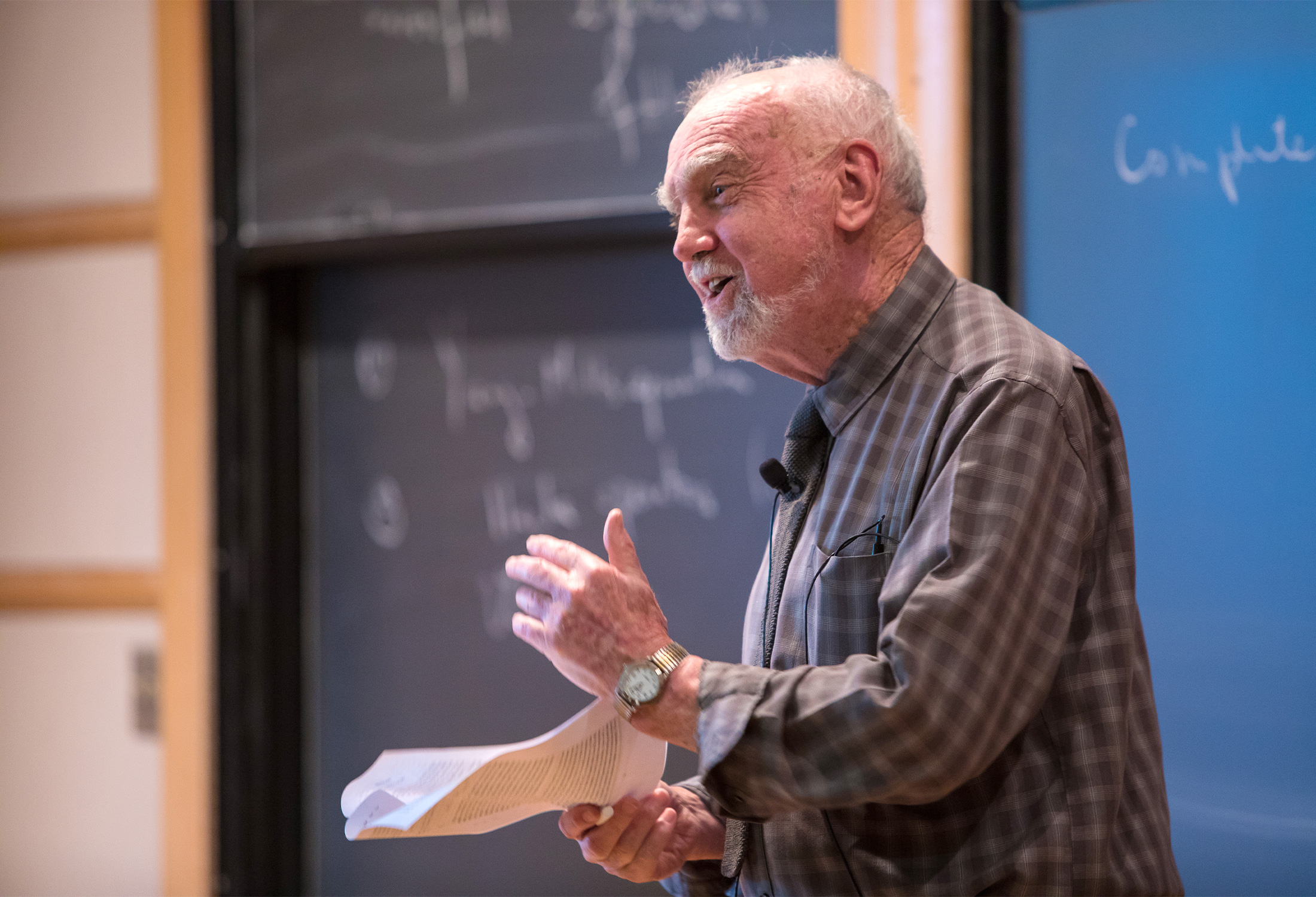
Robert Langlands, who conjectured the influential Langlands correspondence about 50 years ago, giving a talk at the Institute for Advanced Study in Princeton, New Jersey, in 2016.
Dan Komoda/Institute for Advanced Study
Namely, for both Diophantine equations and automorphic forms, there’s a natural way to generate an infinite sequence of numbers. For a Diophantine equation, you can count how many solutions the equation has in each clock-style arithmetic system (for example, in the usual 12-hour clock, 10 + 4 = 2). And for the kind of automorphic form that appears in the Langlands correspondence, you can compute an infinite list of numbers analogous to quantum energy levels.
If you include only the clock arithmetics that have a prime number of hours, Langlands conjectured that these two number sequences match up in an astonishingly broad array of circumstances. In other words, given an automorphic form, its energy levels govern the clock sequence of some Diophantine equation, and vice versa.
This connection is “weirder than telepathy,” Emerton said. “How these two sides communicate with each other … for me it seems incredible and amazing, even though I have been studying it for over 20 years.”
In the 1950s and 1960s, mathematicians figured out the beginnings of this bridge in one direction: how to go from certain automorphic forms to elliptic curves with coefficients that are rational numbers (ratios of whole numbers). Then in the 1990s, Wiles, with contributions from Taylor, figured out the opposite direction for a certain family of elliptic curves. Their result gave an instant proof of Fermat’s Last Theorem, since mathematicians had already shown that if Fermat’s Last Theorem were false, at least one of those elliptic curves would not have a matching automorphic form.
Fermat’s Last Theorem was far from the only discovery to emerge from the construction of this bridge. Mathematicians have used it, for instance, to prove the Sato-Tate conjecture, a decades-old problem about the statistical distribution of the number of clock solutions to an elliptic curve, as well as a conjecture about the energy levels of automorphic forms that originated with the legendary early 20th-century mathematician Srinivasa Ramanujan.
After Wiles and Taylor published their findings, it became clear that their method still had some juice. Soon mathematicians figured out how to extend the method to all elliptic curves with rational coefficients. More recently, mathematicians figured out how to cover coefficients that include simple irrational numbers, such as 3 + $latex \sqrt{2}$.
What they couldn’t do, however, was extend the Taylor-Wiles method to elliptic curves whose coefficients include complex numbers such as i (the square root of −1) or 3 + i or $latex \sqrt{2}$i. Nor could they handle Diophantine equations with exponents much higher than those in elliptic curves. Equations where the highest exponent on the right-hand side is 4 instead of 3 come along for free with the Taylor-Wiles method, but as soon as the exponent rises to 5, the method no longer works.
Mathematicians gradually realized that for these two next natural extensions of the Langlands bridge, it wasn’t simply a matter of finding some small adjustment to the Taylor-Wiles method. Instead, there seemed to be a fundamental obstruction.
They’re “the next examples you’d think of,” Gee said. “But you’re told, ‘No, these things are hopelessly out of reach.’”
The problem was that the Taylor-Wiles method finds the matching automorphic form for a Diophantine equation by successively approximating it with other automorphic forms. But in the situations where the equation’s coefficients include complex numbers or the exponent is 5 or higher, automorphic forms become exceedingly rare — so rare that a given automorphic form will usually have no nearby automorphic forms to use for approximation purposes.
In Wiles’ setting, the automorphic form you’re seeking “is like a needle in a haystack, but the haystack exists,” Emerton said. “And it’s almost as if it’s like a haystack of iron filings, and you’re putting in this magnet so it lines them up to point to your needle.”
But when it comes to complex-number coefficients or higher exponents, he said, “it’s like a needle in a vacuum.”
Going to the Moon
Many of today’s number theorists came of age in the era of Wiles’ proof. “It was the only piece of mathematics I ever saw on the front page of a newspaper,” recalled Gee, who was 13 at the time. “For many people, it’s something that seemed exciting, that they wanted to understand, and then they ended up working in this area because of that.”
So when in 2012, two mathematicians — Frank Calegari of the University of Chicago and David Geraghty (now a research scientist at Facebook) — proposed a way to overcome the obstruction to extending the Taylor-Wiles method, their idea sent ripples of excitement through the new generation of number theorists.
Their work showed that “this fundamental obstruction to going any further is not really an obstruction at all,” Gee said. Instead, he said, the seeming limitations of the Taylor-Wiles method are telling you “that in fact you’ve only got the shadow of the actual, more general method that [Calegari and Geraghty] introduced.”
In the cases where the obstruction crops up, the automorphic forms live on higher-dimensional tilings than the two-dimensional Escher-style tilings Wiles studied. In these higher-dimensional worlds, automorphic forms are inconveniently rare. But on the plus side, higher-dimensional tilings often have a much richer structure than two-dimensional tilings do. Calegari and Geraghty’s insight was to tap into this rich structure to make up for the shortage of automorphic forms.
More specifically, whenever you have an automorphic form, you can use its “coloring” of the tiling as a sort of measuring tool that can calculate the average color on any chunk of the tiling you choose. In the two-dimensional setting, automorphic forms are essentially the only such measuring tools available. But for higher-dimensional tilings, new measuring tools crop up called torsion classes, which assign to each chunk of the tiling not an average color but a number from a clock arithmetic. There’s an abundance of these torsion classes.
For some Diophantine equations, Calegari and Geraghty proposed, it might be possible to find the matching automorphic form by approximating it not with other automorphic forms but with torsion classes. “The insight they had was fantastic,” Caraiani said.
Calegari and Geraghty provided the blueprint for a much broader bridge from Diophantine equations to automorphic forms than the one Wiles and Taylor built. Yet their idea was far from a complete bridge. For it to work, mathematicians would first have to prove three major conjectures. It was, Calegari said, as if his paper with Geraghty explained how you could get to the moon — provided someone would obligingly whip up a spaceship, rocket fuel and spacesuits. The three conjectures “were completely beyond us,” Calegari said.
In particular, Calegari and Geraghty’s method required that there already be a bridge going in the other direction, from automorphic forms to the Diophantine equations side. And that bridge would have to transport not just automorphic forms but also torsion classes. “I think a lot of people thought this was a hopeless problem when Calegari and Geraghty first outlined their program,” said Taylor, who is now at Stanford University.
Yet less than a year after Calegari and Geraghty posted their paper online, Peter Scholze — a mathematician at the University of Bonn who went on to win the Fields Medal, mathematics’ highest honor — astonished number theorists by figuring out how to go from torsion classes to the Diophantine equations side in the case of elliptic curves whose coefficients are simple complex numbers such as 3 + 2i or 4 − $latex \sqrt{5}$i. “He’s done a lot of exciting things, but that’s perhaps his most exciting achievement,” Taylor said.
Scholze had proved the first of Calegari and Geraghty’s three conjectures. And a pair of subsequent papers by Scholze and Caraiani came close to proving the second conjecture, which involves showing that Scholze’s bridge has the right properties.
It started to feel as if the program was within reach, so in the fall of 2016, to try to make further progress, Caraiani and Taylor organized what Calegari called a “secret” workshop at the Institute for Advanced Study. “We took over the lecture room — no one else was allowed in,” Calegari said.
After a couple of days of expository talks, the workshop participants started realizing how to both polish off the second conjecture and sidestep the third conjecture. “Maybe within a day of having actually stated all the problems, they were all solved,” said Gee, another participant.
The participants spent the rest of the week elaborating various aspects of the proof, and over the next two years they wrote up their findings into a 10-author paper — an almost unheard-of number of authors for a number theory paper. Their paper essentially establishes the Langlands bridge for elliptic curves with coefficients drawn from any number system made up of rational numbers plus simple irrational and complex numbers.
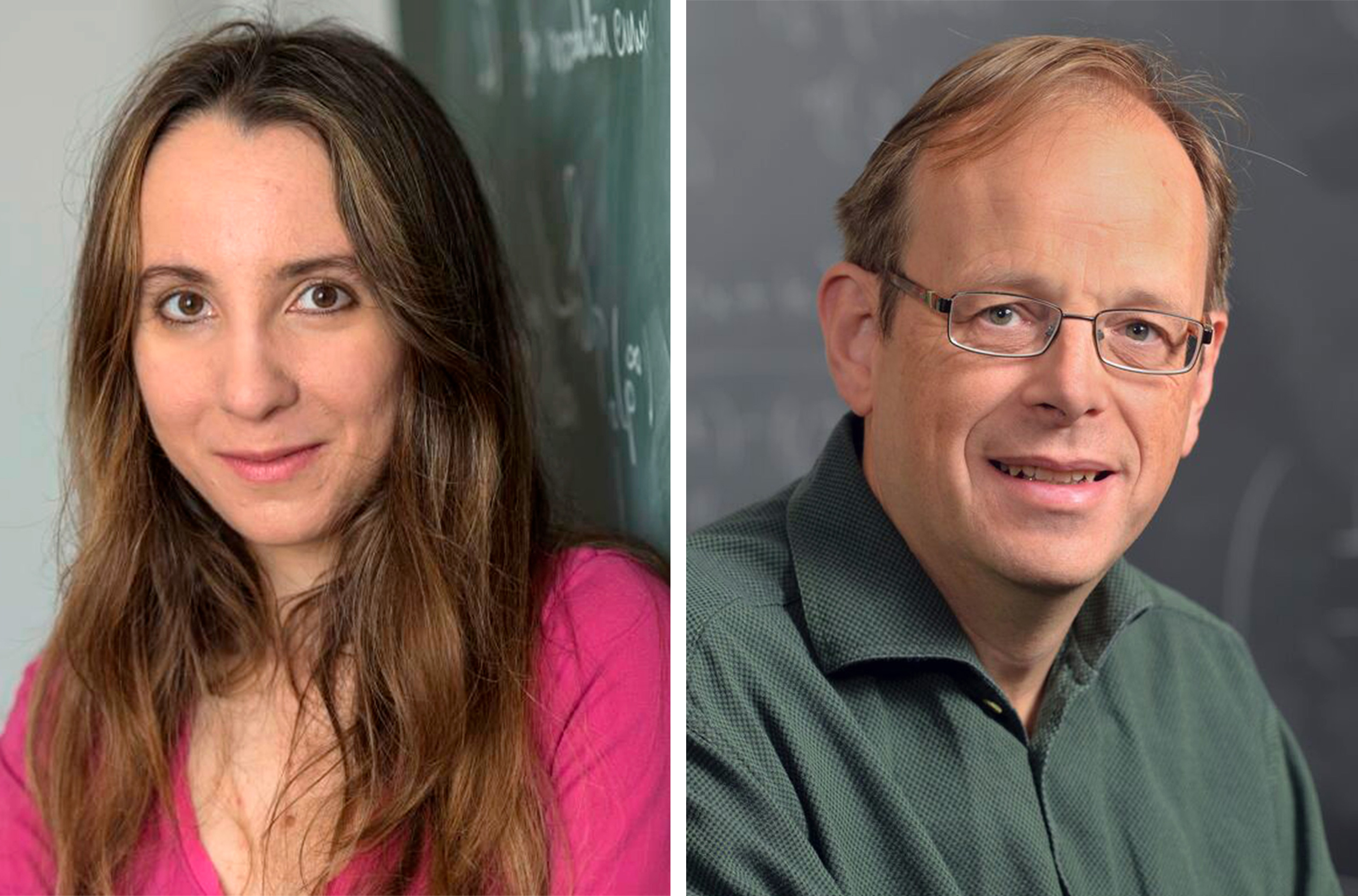
In the fall of 2016, Ana Caraiani and Richard Taylor convened a “secret” workshop at the Institute for Advanced Study in 2016 that quickly resolved two outstanding problems and led to a major 10-author paper.
Hausdorff Center for Mathematics; Rod Searcey
“The plan in advance [of the workshop] was just to see how close one could get to proving things,” Gee said. “I don’t think anyone really expected to prove the result.”
Extending the Bridge
Meanwhile, a parallel story was unfolding for extending the bridge beyond elliptic curves. Calegari and Gee had been working with George Boxer (now at the École Normale Supérieure in Lyon, France) to tackle the case where the highest exponent in the Diophantine equation is 5 or 6 (instead of 3 or 4, the cases that were already known). But the three mathematicians were stuck on a key part of their argument.
Then, the very weekend after the “secret” workshop, Vincent Pilloni of the École Normale Supérieure put out a paper that showed how to circumvent that very obstacle. “We have to stop what we’re doing now and work with Pilloni!” the other three researchers immediately told each other, according to Calegari.
Within a few weeks, the four mathematicians had solved this problem too, though it took a couple of years and nearly 300 pages for them to fully flesh out their ideas. Their paper and the 10-author paper were both posted online in late December 2018, within four days of each other.
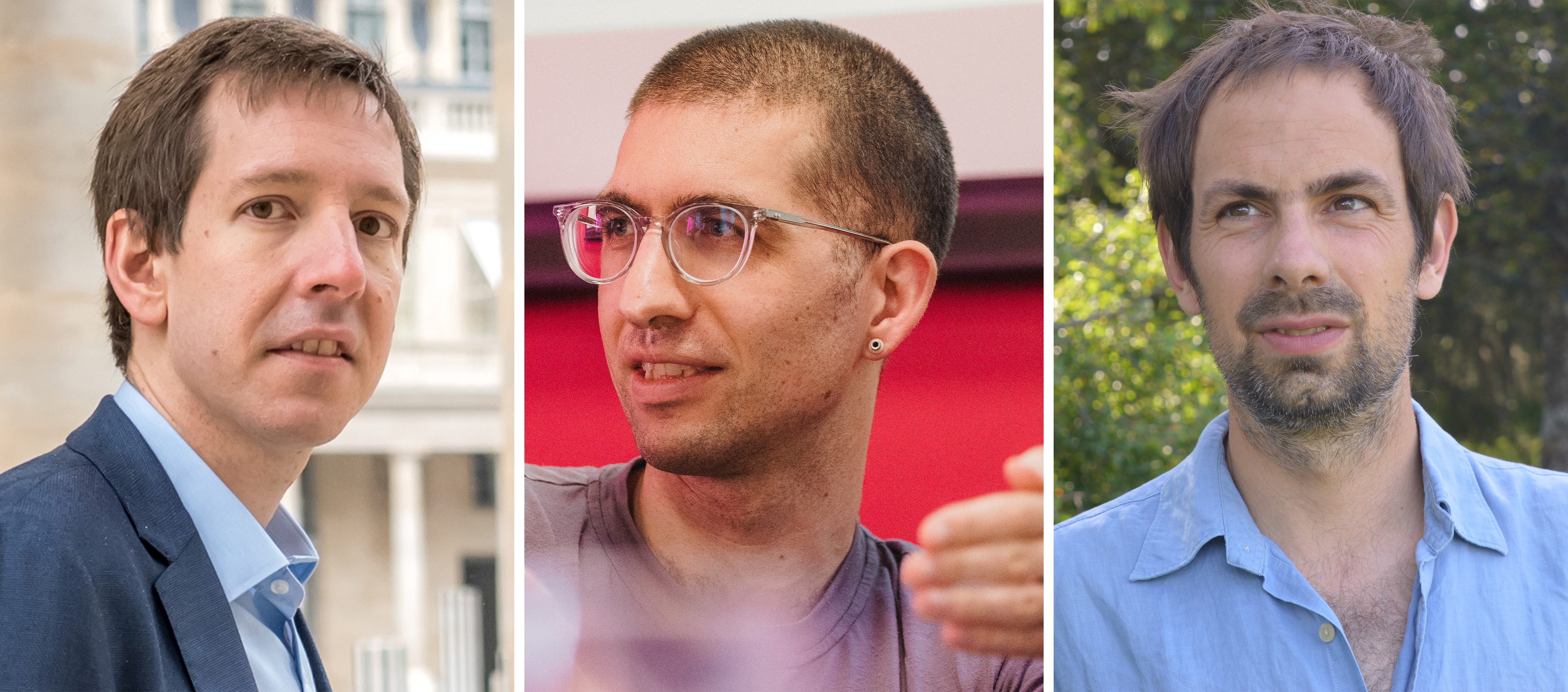
Soon after the secret workshop at the IAS, Frank Calegari (left), Toby Gee (center) and Vincent Pilloni, working with George Boxer (not pictured), found a way to extend the Langlands bridge beyond elliptic curves.
Frank Calegari, University of Chicago; Courtesy of Toby Gee; Arnold Nipoli
“I think they’re pretty huge,” Emerton said of the two papers. Those papers and the preceding building blocks are all “state of the art,” he said.
While these two papers essentially prove that the mysterious telepathy between Diophantine equations and automorphic forms carries over to these new settings, there’s one caveat: They don’t quite build a perfect bridge between the two sides. Instead, both papers establish “potential automorphy.” This means that each Diophantine equation has a matching automorphic form, but we don’t know for sure that the automorphic form lives in the patch of its continent that mathematicians would expect. But potential automorphy is enough for many applications — for instance, the Sato-Tate conjecture about the statistics of clock solutions to Diophantine equations, which the 10-author paper succeeded in proving in much broader contexts than before.
And mathematicians are already starting to figure out how to improve on these potential automorphy results. In October, for instance, three mathematicians — Patrick Allen of the University of Illinois, Urbana-Champaign, Chandrashekhar Khare of the University of California, Los Angeles and Jack Thorne of the University of Cambridge — proved that a substantial proportion of the elliptic curves studied in the 10-author paper do have bridges that land in exactly the right place.
Bridges with this higher level of precision may eventually allow mathematicians to prove a host of new theorems, including a century-old generalization of Fermat’s Last Theorem. This conjectures that the equation at the heart of the theorem continues to have no solutions even when x, y and z are drawn not just from whole numbers but from combinations of whole numbers and the imaginary number i.
The two papers carrying out the Calegari-Geraghty program form an important proof of principle, said Michael Harris of Columbia University. They’re “a demonstration that the method does have wide scope,” he said.
While the new papers connect much wider regions of the two Langlands continents than before, they still leave vast territories uncharted. On the Diophantine equations side, there are still all the equations with exponents higher than 6, as well as equations with more than two variables. On the other side are automorphic forms that live on more complicated symmetric spaces than the ones that have been studied so far.
“These papers, right now, are kind of the pinnacle of achievement,” Emerton said. But “at some point, they will just be looked back at as one more step on the way.”
Langlands himself never considered torsion when he thought about automorphic forms, so one challenge for mathematicians is to come up with a unifying vision of these different threads. “The envelope is being expanded,” Taylor said. “We’ve to some degree left the path laid out by Langlands, and we don’t quite know where we’re going.”
This article was reprinted on Wired.com.