Cells Blaze Their Own Trails to Navigate Through the Body
Introduction
Even when it’s not apparent, cells in our tissues and organs are constantly on the move. In fact, the ability of cells to get where they need to go is essential to our health and survival. Skin cells migrate to heal wounds. Immune system cells migrate to fight infections.
“Every day, you look at your body and it’s not changing much,” said Peter Devreotes (opens a new tab), a professor of cell biology at the Johns Hopkins University School of Medicine. “But the cells within it are migrating constantly.”
It starts from the earliest stages of life. When we are embryos just a few weeks old, a special population of “neural crest” cells in our back suddenly spreads through the body to become a wide range of essential tissues — bones, cartilage and nerves in the face, tendons, pigment cells in the skin, parts of the heart and more.
But how do these cells know where to go? Studies long suggested that they were following chemical trails to their routes. Biologists traditionally saw these chemical gradients as simple and the cells as mere followers: Like dogs trotting toward the scent of food, the cells sensed the gradient and followed the stream of signals back to the source. Countless examples of this have been found among bacteria and other cells navigating through the wild, as well as inside larger organisms. When you nick your skin, for instance, the tissue around the cut releases a cloud of molecules that attract immune cells nearby. The immune cells crawl toward it and stave off infection.
Yet scientists also came to understand that this system can’t sustain many of the migrations that unfold in the body. The structure of simple passive gradients is too fragile and too easily disrupted. Simple gradients like these don’t always reach far enough to guide cells’ lengthier journeys, and they may dissipate too quickly to maintain migrations that take longer. Raising the sensitivity of the cells might seem like a way to offset those problems, but then cells might often be too flooded with signals to sense where they come from. For a simple gradient to work, it has to be perfect, and nothing can go awry. But in reality, cells must find a way to navigate under all kinds of conditions.
Now researchers have discovered another crucial part (opens a new tab) of the answer, one that helps explain how cells are directed to their destinations in the neural crest migration and probably other movements as well. The new work shows that in addition to using chemical cues, neural crest cells “feel” their way through the body, creating patterns of physical tension in the surrounding tissue that point them the right way. In effect, the cells create the signals that they use to steer themselves.
Finding this navigation mechanism doesn’t just clarify how neural crest cells make their vital migration. It also further validates an idea that has been gathering force in just the last few years: that “self-generated gradients” are essential to cell migrations, and that these gradients can be built from all kinds of factors — not just chemicals.
How Cells Conquer Mazes
Robert Insall (opens a new tab) of the University of Glasgow realized something was wrong with the traditional explanation when his team was running the control for an experiment a few years ago. To show that cell migration requires an existing gradient, he evenly spread lysophosphatidic acid (LPA), a chemical attractant that cells commonly use as a signaling molecule, across the bottom of a container. Then he deposited cancer cells on one side of the container. Without a highly concentrated source of the signal to guide them, he expected that if the cells moved much at all, it would be aimless.
Instead, the cells surprised him by crawling to the other end of the chamber even without the gradient as a clear trail. The cells were breaking down the LPA around them, and then migrating to where they sensed more.
“You’re doing this control, which is supposed to show that you understand what’s going on,” Insall said. “But it turns out you don’t understand at all.”
Insall later learned that this simple mechanism had been unearthed half a century previously. For a 1966 paper in Science, Julius Adler of the University of Wisconsin, Madison watched Escherichia coli cells make their way across a petri dish filled evenly with nutrients and oxygen. The bacteria used up the fuel near them so there was less nearby and more on the other side — a good reason to migrate. He wrote that “the bacteria create a gradient.” Though Adler didn’t use the term “self-generated,” he completely understood that the gradient was exactly that, Insall said. “And then the whole idea, the moral, was essentially forgotten for the next 50 years.”
Insall and his team began experiments to better understand self-generated gradients. They engineered minuscule vessels with intricate passageways and recorded cells clambering through them. And they simulated self-generated gradients in models that turned out to closely mirror cells’ real-world movements.
In 2016 Insall and his colleagues proposed that cells can use self-generated gradients to steer themselves (opens a new tab). But that wasn’t all: They also showed that with these gradients, the cells could solve complicated mazes (opens a new tab), including a miniature imitation of the famous trapezoidal hedge maze that King William III built at Hampton Court in England. Groups of cells would break down the LPA in dead ends, sense the dearth, and try another path. Or if there was too much LPA flooding the area, cells could break down the buildup until they could sense the direction of the gradient again. The cells could follow incoming molecules to their source, even if it was around a corner or far away.
In one round of experiments, a glitch in the maze opened up a shortcut to the source of LPA. “We watched in amazement, really, as we loaded all these cells in and they instantly saw the shortcut,” Insall said. Cells snuck through, like shoppers taking the shortcuts at Ikea that spare them a trip through the sofa section, he added.
“This alternative mechanism for guiding cells … confers incredible ability onto these cells,” said Darren Gilmour (opens a new tab), a professor of molecular life sciences at the University of Zurich. Self-generated gradients explain cell movements that can’t be explained otherwise. “Wherever there is a signal, the cells can sculpt it to keep moving directionally,” he added. “It’s just such an elegant mechanism.”
Self-generated gradients have now made sense of perplexing behavior in cancer cells, fish embryos, immune cells, bacteria, slime mold, and more — and findings are accumulating rapidly. “People are opening their eyes, and it’s now seen everywhere, suddenly,” said Jonna Alanko (opens a new tab), a postdoc at the Institute of Science and Technology Austria (opens a new tab). “I’m pretty sure that this is only the tip of the iceberg.”
Insall expects that while simple gradients are still part of the picture, most chemically guided cell migration uses self-generated gradients. “We find examples wherever we look for them,” he said.
A Moving Soft Spot
Much of the research to date on self-generated gradients has looked at chemical signals, but cells can create gradients in other physical attributes, too, including mechanical properties. The recent paper (opens a new tab) analyzing migrating neural crest cells revealed a self-generated gradient of stiffness, to the authors’ surprise.
To grasp how neural crest cells navigate, Adam Shellard (opens a new tab) of University College London tested the rigidity of their surroundings. He meticulously probed the tissues within frog embryos that measured only a millimeter across. Pressing here and there to record stiffness levels, he noticed a gradient with one softer area amid more rigid tissues. Intriguingly, the soft spot didn’t stay still. More experiments revealed that the migrating neural crest cells were softening the extracellular matrix, or protein scaffolding around neighboring cells, as they traveled.
Merrill Sherman/Quanta Magazine
But even though the neural crest cells cause this softening in their surroundings, they don’t want to stay in it — they respond by shifting to the stiffer areas up ahead. Perhaps the cells do so because, by analogy, it’s easier to walk on pavement than sand, explained Roberto Mayor (opens a new tab) of University College London, the other author of the study.
The researchers already knew that the “placode” cells in front of the migrating cells produces a chemical attractant to help draw the cells forward. These placode cells are repelled by the touch of the neural crest cells, so they run in the opposite direction. The newly discovered mechanical gradient works in tandem with the chemical cues to drive the migration of the neural crest cells forward by a “chase and run” mechanism.
“No one thought it could really be true, or thought there was a means for that to work, but it seems to be,” Shellard said.
When Insall read the paper, it made perfect sense to him. “It’s very satisfying. You figure, ‘yes, that must happen,’” he said.
The idea is catching on. Once the paper was published, Mayor’s inbox was flooded with messages from other researchers about the same kind of mechanism that seemed to be at work in embryos, immune cells and cancer. Self-generated stiffness gradients will turn out to be common, Mayor predicted. “There are many papers coming out very soon that will show this.”
How Gradients Even Out Irregularities
Some researchers have been sussing out the reasons self-generated gradients work so well and are so impressively resilient to disruptions (opens a new tab).
Research led by Sujit Datta (opens a new tab), an assistant professor of chemical and biological engineering at Princeton University, has illustrated just how robust self-generated gradients can be. In a recent paper in eLife (opens a new tab), Datta’s team 3D-printed squiggles of E. coli into gels — “basically like ball pits for cells,” he said. No matter how squiggly the lines were, the migrating cells always smoothed out into an even band as they spread outward into the gel.
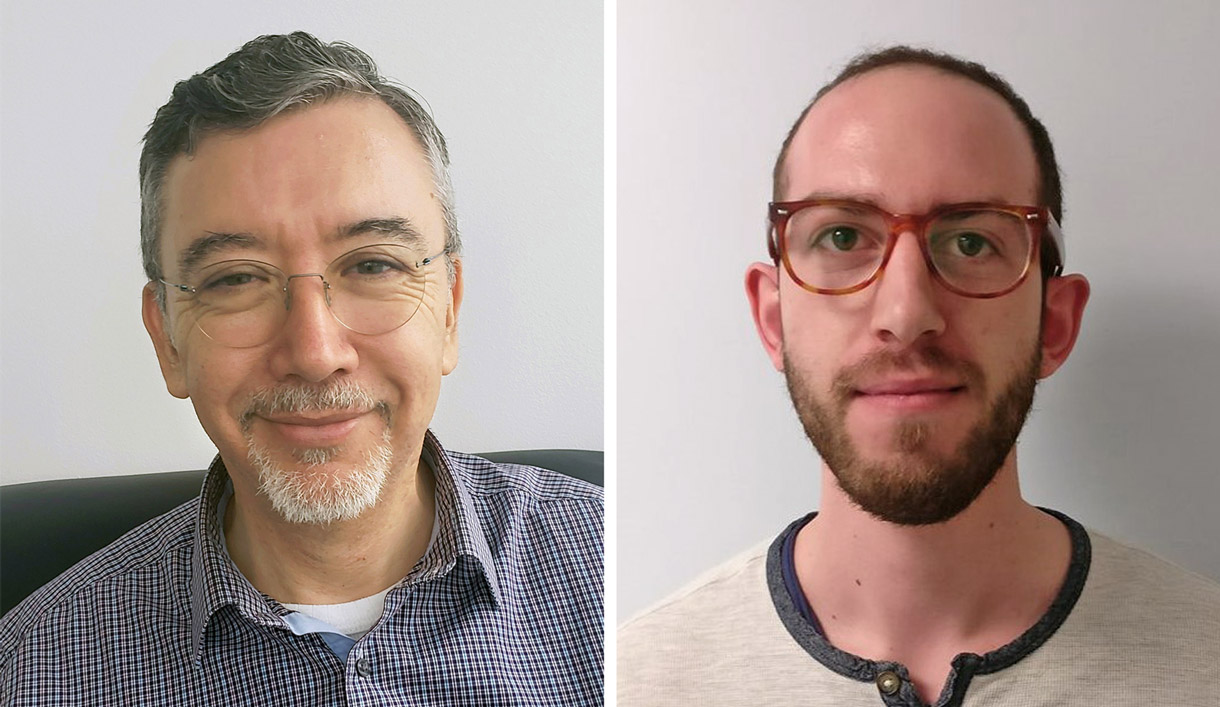
Recent work by researchers Roberto Mayor (left) and Adam Shellard (right) of University College London showed that some cells direct their migrations by creating gradients in the stiffness of their surroundings.
Roberto Mayor (left). Adam Shellard (right)
Self-generated gradients explained why. The bacteria atop the “hills” of the squiggles were closer to a region of gel filled with nutrients, and that abundance saturated their sensors. They didn’t begin to spread outward until after they broke down all the local nutrients and could sense which way to go for more. In the “valleys” of the squiggles, however, the bacteria had fewer nutrients nearby. They were able to set their course and take off earlier. This headstart let them catch up to the bacteria already on the top of the hills, which flattened out the advancing front of the migrating cells.
Datta observed in a preprint (opens a new tab) (now in press at Physical Review Letters) that this same principle may be true for other kinds of gradients as well — including the stiffness gradient Mayor and Shellard mapped in frog embryos. Different gradients are likely to withstand these disruptions, too. The gradients may help to smooth out important processes in development and healing so that they are not easily thrown off by disruptions.
Insall speculates that the robustness of self-generated gradients could affect the prospects for some proposed cancer therapies. He thinks that treatments aiming to curb cancer by impeding the self-generated gradients it follows through the body are unlikely to succeed: They might delay its spread but the cells are too likely to reestablish the gradients. But flipping this strategy on its head might work better: Treatments could establish competing gradients so that cells spread to destinations in the body where they might be less harmful and more vulnerable.
The concept of self-generated gradients is not significant just because it explains the abilities of cells on the move. Biologists sometimes think about cells as though their behavior were predetermined by their genes, according to Darren Gilmour (opens a new tab), a professor of molecular life sciences at the University of Zurich. But the new navigational discoveries show that groups of cells are less like soldiers following precise orders and more like soccer players. “When the ball comes to them, what do they do?” he asked: They make decisions on the fly and adapt to changing surroundings.
“We’re realizing that there’s a lot more control at the level of the cells,” Gilmour said. “And they make the decisions together.”