The Mysterious Forces Inside the Nucleus Grow a Little Less Strange
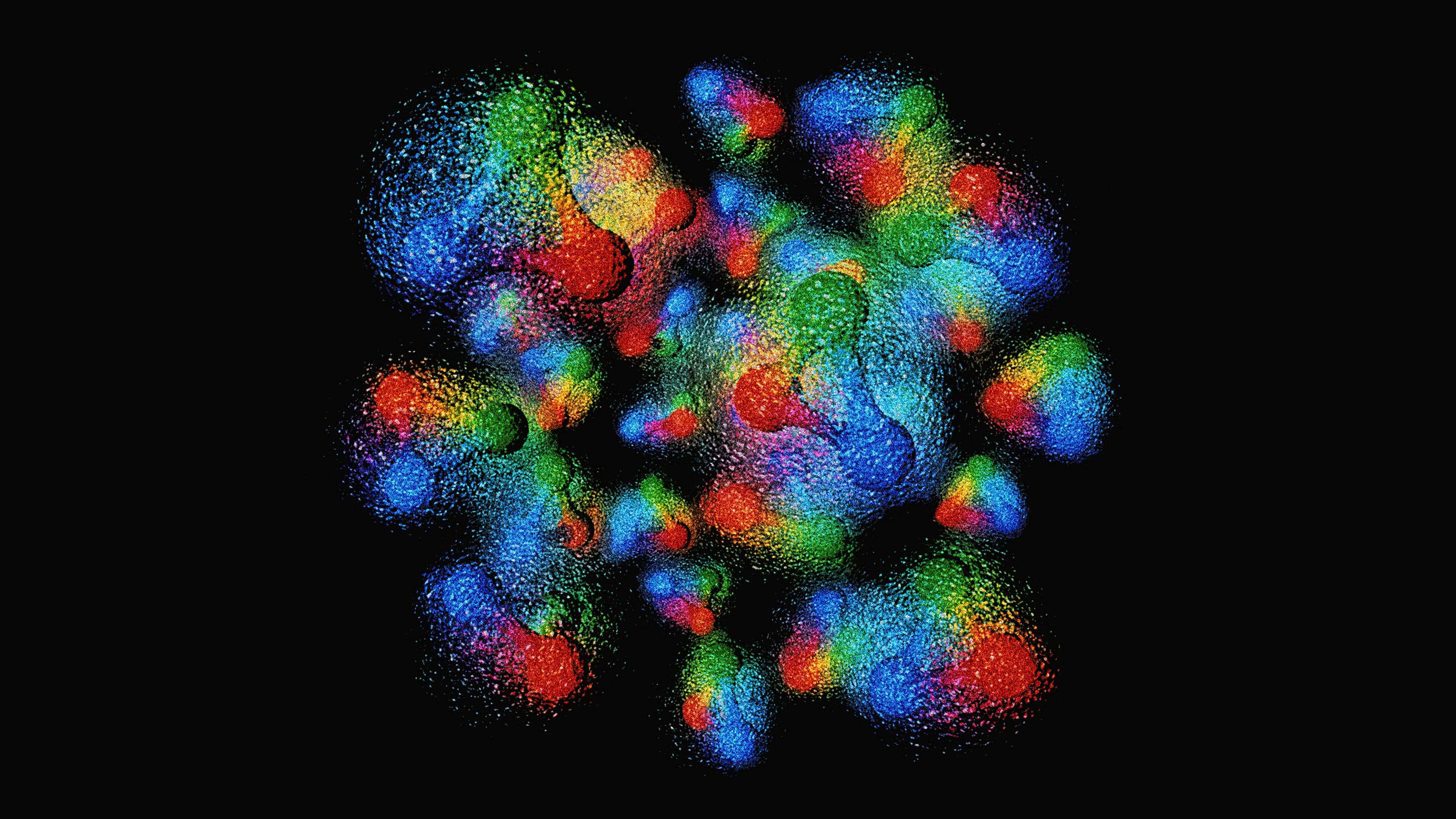
In 1935, Hideki Yukawa explained why protons and neutrons — particles made of quarks — stick together. Only now do physicists have the tools to investigate how rarer groupings of quarks interact.
ArSciMed / Science Source
Introduction
Billions of times each minute, the Large Hadron Collider (LHC) smashes protons together, unleashing a maelstrom of energy that crystallizes into more protons, neutrons, and less familiar cousins of the nuclear particles. Some particles encounter each other as they flee the scene. What happens next — whether a given pair pulls together or pushes apart — physicists generally can’t say.
Theorists worked out how the particles inside protons and neutrons act more than 50 years ago. But these particles, known as quarks, never appear alone, and the hard-won theory of their force — the strong force — fails to predict the behavior of groups of quarks, the objects that actually make up our bodies and appear in particle colliders.
“This is, if you want, the frontier of nuclear physics,” said Laura Fabbietti, a physicist at the Technical University of Munich, “understanding [these] interactions from first principles.”
After decades of work, powerful ways of spying on “hadrons” — particles made of multiple quarks — are finally reaching maturity. Supercomputers can now calculate the force between certain digital hadrons. And researchers at the LHC are pioneering a new method, known as femtoscopy, that can directly detect tremors between fleeting hadrons induced by the strong force. The research is revealing novel aspects of nature’s most inscrutable force.
“All of a sudden, we can test for the first time the strong interaction” between any pair of hadrons, said Fabbietti, one of femtoscopy’s developers.
The Enigmatic Nucleus
The core of the atom has challenged physicists since the 1930s, when leaders in the field including Enrico Fermi and Werner Heisenberg struggled to reconcile several bizarre observations. One was the fact that the nucleus even existed. Take the helium nucleus, where two protons nestle together just a couple of femtometers (millionths of a billionth of a meter) apart. At that distance, the two positive charges should blow the nucleus apart with 20 pounds of force. Yet stable helium atoms abound. Electromagnetism controls the atom, but the nucleus seems to play by different rules.
A relatively unknown Japanese physicist, Hideki Yukawa, hit on a major piece of the nuclear puzzle in 1935.
If some mighty force was holding the nucleus together, it was a weird one. The massless photon carries the electromagnetic force far and wide, but protons and neutrons need close contact to stick. Yukawa’s main insight was that this short range results from the new force’s particle having a mass that limits its mobility; he calculated that it should have 200 times the heft of the electron. Physicists discovered the pi meson or “pion” in cosmic rays in 1947, with a mass just one-third larger than Yukawa had predicted. His Nobel Prize came two years later.
He “was the first person who predicted the existence of some new particle,” said Tetsuo Hatsuda, a nuclear physicist and program director of the RIKEN institute in Japan. “That was the birth of particle physics.”
The pion proved the first of a deluge of new particles. Patterns in this growing menagerie led theorists to conclude that quarks come in six varieties and bind so tightly that they always exist in groups. Today, physicists know of more than 300 unique hadrons.
Theorists worked out how the strong force governs quarks — a theory known as quantum chromodynamics, or QCD — in the 1970s. But frustratingly, it just doesn’t provide all the answers.
QCD depicts quarks as swapping flurries of force-carrying “gluons” with an intensity that grows with distance, like the tension in an elastic band. When particles slam together, as they do in a particle collider, quarks get so close together that the elastic goes slack. In these situations, QCD works well. But under ordinary circumstances, the elastic stretches out and engages, and the mathematics of QCD break down. This limitation makes the real-world behavior of hadrons a mystery.
“This is the only [incalculable] piece in the Standard Model of elementary particle physics,” said Tetsuo Hyodo, a physicist at Tokyo Metropolitan University.
Hadron High Jinks
Even without a solvable theory, two techniques are increasingly helping physicists crack the particles’ cryptic conduct.
The first is to outsource the problem to supercomputers. Researchers set up a digital laboratory as follows: They divide space into a grid, and time into a series of discrete moments. They stick quarks where the grid lines intersect, and gluons on the links between them. They then calculate what happens frame by frame in a way that would be impossible for smooth space and time.
In 2007, Hatsuda’s research group used this “lattice QCD” approach to simulate pairs of protons or neutrons as more realistic clouds of quarks and gluons, rather than Yukawa-esque points. They confirmed that when the protons or neutrons are about one proton width apart, they really do attract as if by exchanging pions. “In some sense, Yukawa is confirmed on the basis of QCD,” said Hatsuda. The group also went beyond Yukawa’s theory and proved that attraction turns into repulsion when the particles get even closer.
Recently, the team has been whipping up virtual encounters between a proton or neutron (containing lighter “up” and “down” quarks) and an “omega” hadron made from three heavier “strange” quarks. They found in 2019 that the pair of hadrons tug on each other near and far. And in 2020, the collaboration calculated that a pair of “lambdas” (an up quark, a down quark and a heavier quark) attract weakly. These results represent some of the first hints at how heavier hadrons, which tend to decay in a flash, affect each other.
In parallel, LHC researchers have started harnessing the ALICE experiment to track real hadrons. Proton collisions produce a burst of hadrons, many of which decay into other particles. ALICE researchers pick through this wreckage to find signs of desired hadron pairings. They compare hadron couples that moved together along similar paths with those that went in different directions. The goal is to uncover signs of how nearby hadrons might attract or repel each other. The technique can detect hadronic twitches as small as a single femtometer.
“The beauty is that you can apply this technique to hadrons which are very rare and unstable,” said Fabbietti, who leads the femtoscopy group within the ALICE collaboration. “Normally there aren’t other chances to let two particles talk to each other and see what they say.”
The collaboration detailed their femtoscopy method in Nature in 2020. Last October, they unveiled a measurement of a largely unknown interaction, one between a proton and a phi meson (which consists of a strange quark and its antiquark). Experimentalists normally lean on theoretical data from lattice QCD to interpret their data, but so little work has been done on phi mesons that they had to go back to Yukawa’s 1935 theory.
“For proton-phi there was nothing,” said Emma Chizzali, the graduate student who led the data analysis.
Protons and phi mesons that came close to each other appeared to attract, the ALICE collaboration concluded from roughly 100,000 pairings. Yet the attraction was only one-tenth as strong as that between protons and neutrons.
The experiment is “very exciting,” said Hatsuda. His team is currently checking the result with lattice QCD.
From the LHC to Neutron Stars
While hadrons containing strange quarks decay quickly at the LHC, they may exist as long-term residents of neutron stars, where immense pressures could stabilize strange proton variants known as “hyperons.” These hyperons would swap phi mesons instead of pions, which some theorists have proposed could stiffen the dead stars. But ALICE’s result suggests that the strange interactions are too feeble to matter.
“If there are hyperons inside the neutron stars,” Fabbietti said, “their interactions can be completely neglected.”
Hyodo hopes that comprehensive knowledge of which two- and three-quark particles stick together could explain another mystery — why groupings of four or five quarks are so rare. Physicists have cataloged hundreds of quark duos and trios, but just a handful of tetraquarks and pentaquarks.
To that end, ALICE has been sifting through a billion or so collisions that took place between 2016 and 2018. Starting this spring, however, an upgrade to the LHC will let them take data 100 times faster. Over the next decade, Fabbietti expects to measure the mingling of rarer hadrons containing even heavier quarks.
“We are building this puzzle,” she said, “trying to measure all of them.”