The Materials Scientist Who Studies the Innards of Exoplanets
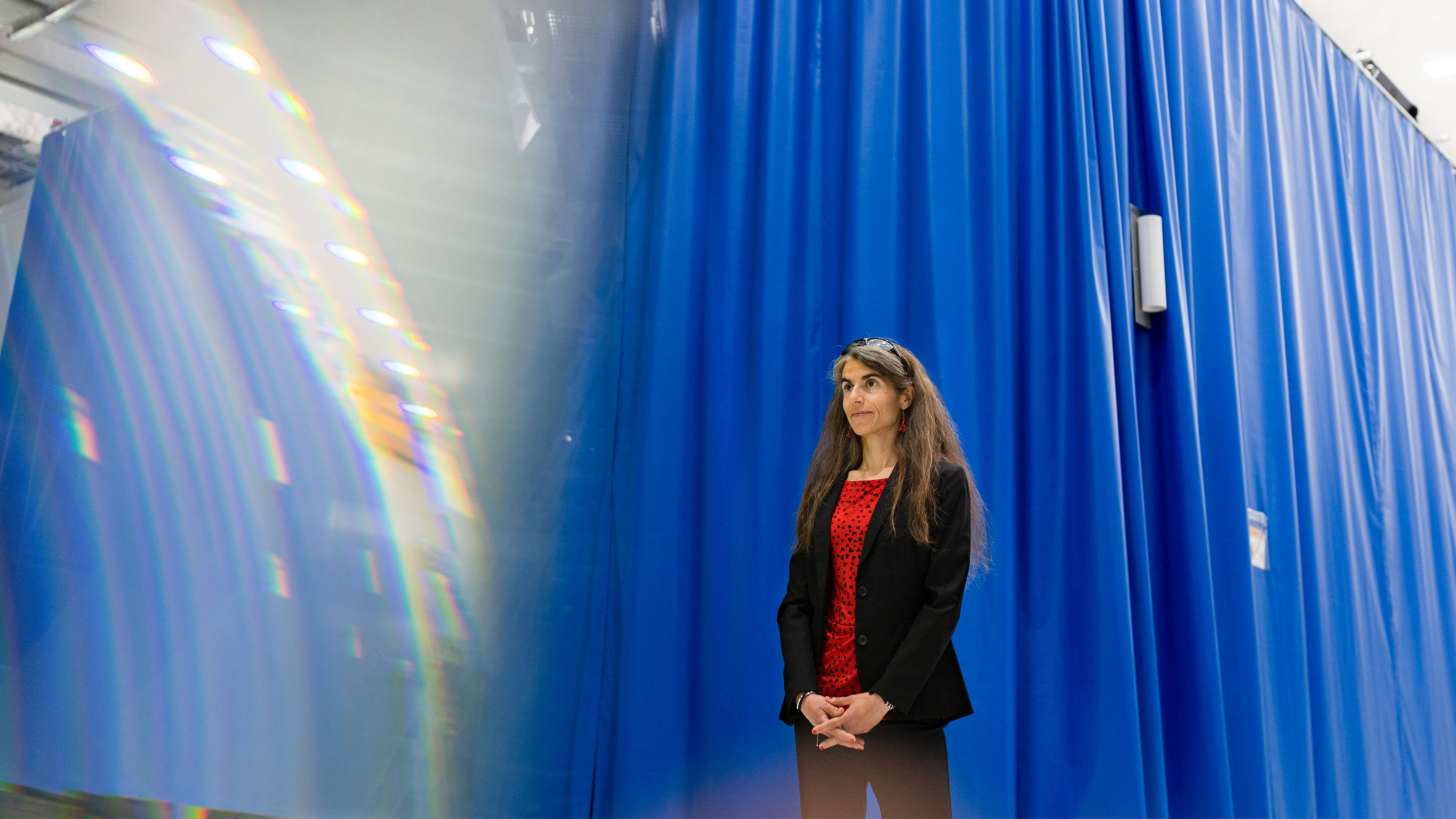
Federica Coppari at the National Ignition Facility in Livermore, California.
Constanza Hevia for Quanta Magazine
Introduction
Out in the vast universe, unknown billions of strange worlds drift around other stars. Many of them are quite unlike anything in our solar system. While astronomers hope to use immense upcoming observatories to get a better look at their outsides, Federica Coppari has been using the world’s largest laser to investigate their insides.
Coppari compresses familiar substances, including rocks and water, into new forms. Her work has yielded insights into the inner workings of frozen giants such as Uranus and Neptune, as well as the potential habitability of super-Earths — rocky planets that dwarf our own.
Two years ago, she and her colleagues confirmed the existence of “superionic ice,” a hot, black form of the normally chilly substance. This long-sought phase may be among the most common forms of ice in the universe, found inside ice giants both in our own solar system and elsewhere. The work has helped solve puzzles about such worlds’ magnetic fields.
It’s here, where the terrestrial and the celestial meet, that Coppari thrives. “How our experiments in the laboratory can say something about what’s going on up there — that’s fascinating,” she said.
Like many kids, Coppari went through a period where she wanted to be an astronaut. But guided by her teachers, she realized that she was more interested in figuring out “why things are the way they are, and why things happen the way they do.” That quest took her out of Appignano, the tiny town in Italy where she grew up, to Paris and then to the U.S., where she is currently a scientist at Lawrence Livermore National Laboratory in California.
There, she works with the National Ignition Facility, or NIF, a laser the size of three football fields. NIF was originally intended to help maintain the country’s stockpile of thermonuclear weapons and to pursue the dream of harnessing fusion for energy. Coppari uses the beam’s intense blasts to conduct laser-driven compression experiments. Each laser “shot” may last only a nanosecond, but it’s capable of recreating the pressure at the core of an enormous planet.
More recently, she’s been part of a team that used the University of Rochester’s Omega Laser Facility to compress samples of iron oxide — one of the main constituents of our planet’s mantle — up to 7 million times Earth’s atmospheric pressure. Coppari and her colleagues were able to glean information that could be used to model super-Earth interiors, helping to figure out details about their magnetic fields, crustal movements, and perhaps even their suitability for living things.
Quanta Magazine spoke with Coppari about how the terrestrial can provide insight into the celestial. The interview has been condensed and edited for clarity.
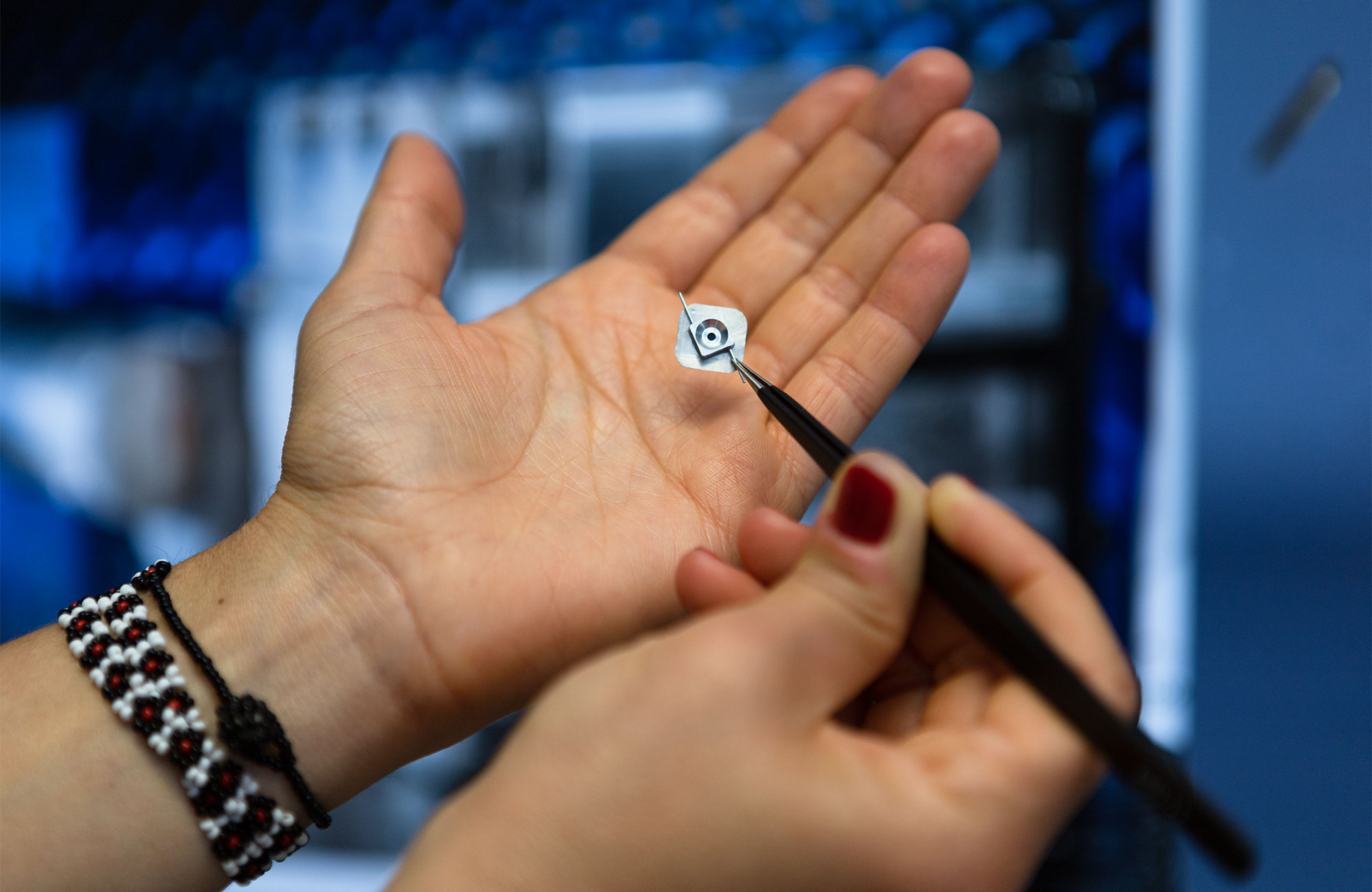
The target of one of Coppari’s experiments. Water enters through the two small fill tubes, then the tubes are cut and sealed.
Constanza Hevia for Quanta Magazine
Where did you grow up?
It’s a small, very quiet town — like 4,000 people — in the center of Italy, midway between the mountains and the Adriatic Sea. If you hadn’t grown up in such a place, you’d say it’s cute. But growing up there, you see what’s not so exciting about being in a town where everyone knows everything about everyone. Early on during my teenage period, I felt like I had to see what was around.
I went to college not too far from where I grew up. Then I again had this feeling that I wanted to see a little further away. The opportunity came to work with a professor who had a joint position in Camerino, Italy, and in also in Paris, France. I was able to spend a few months in Paris in a laboratory for mineralogy and high-pressure physics, and that’s basically how I started working in high-pressure physics.
What sparked your attraction to it?
In everyday life, you experience the changes that materials undergo when they’re heated — we see water freezing and boiling. But the effects of pressure are more tricky to grasp. It was fun to see how easy it is to change a material’s properties just by applying a little pressure.
Did Paris satisfy the need to see the world?
A little bit. In Paris, there’s everything you can look for: culture, entertainment, theater, music. But also a great laboratory, and that’s why I stayed. I got to spend four years in Paris. I have good memories, although after a while I got tired of the big city, because you feel like you’re always busy. Plus, you have this ideal picture of Paris as a beautiful city, but I think in a year I only counted 10 days of full sun. The rest, it’s cloudy and gray.
How did you end up at Livermore?
My Ph.D. adviser in Paris knew one of the research scientists at Livermore who had started working with dynamic compression — using lasers to generate high temperatures and pressures. For me it was sort of a change in field. I had to learn about shock physics, which I had never really studied. But I thought it wouldn’t be too big of a jump and I gave it a try. My first time in the U.S. was quite overwhelming. I liked it even though, at that time, I didn’t fully understand what I was getting into.
What do you mean?
I mean how things work when you’re using lasers to compress materials. They told me, “We’re using lasers. You get to squeeze materials, and we’re trying to combine these compression techniques with X-ray diagnostics, so that you can see in situ what the compression does to the material.” It sounds easy enough but when you dig into the problem, it’s a little more complicated. That’s what I didn’t get at the beginning: I had to learn a whole new physics, and a whole new diagnostics, and a new way of analyzing data, and new ways of preparing samples.
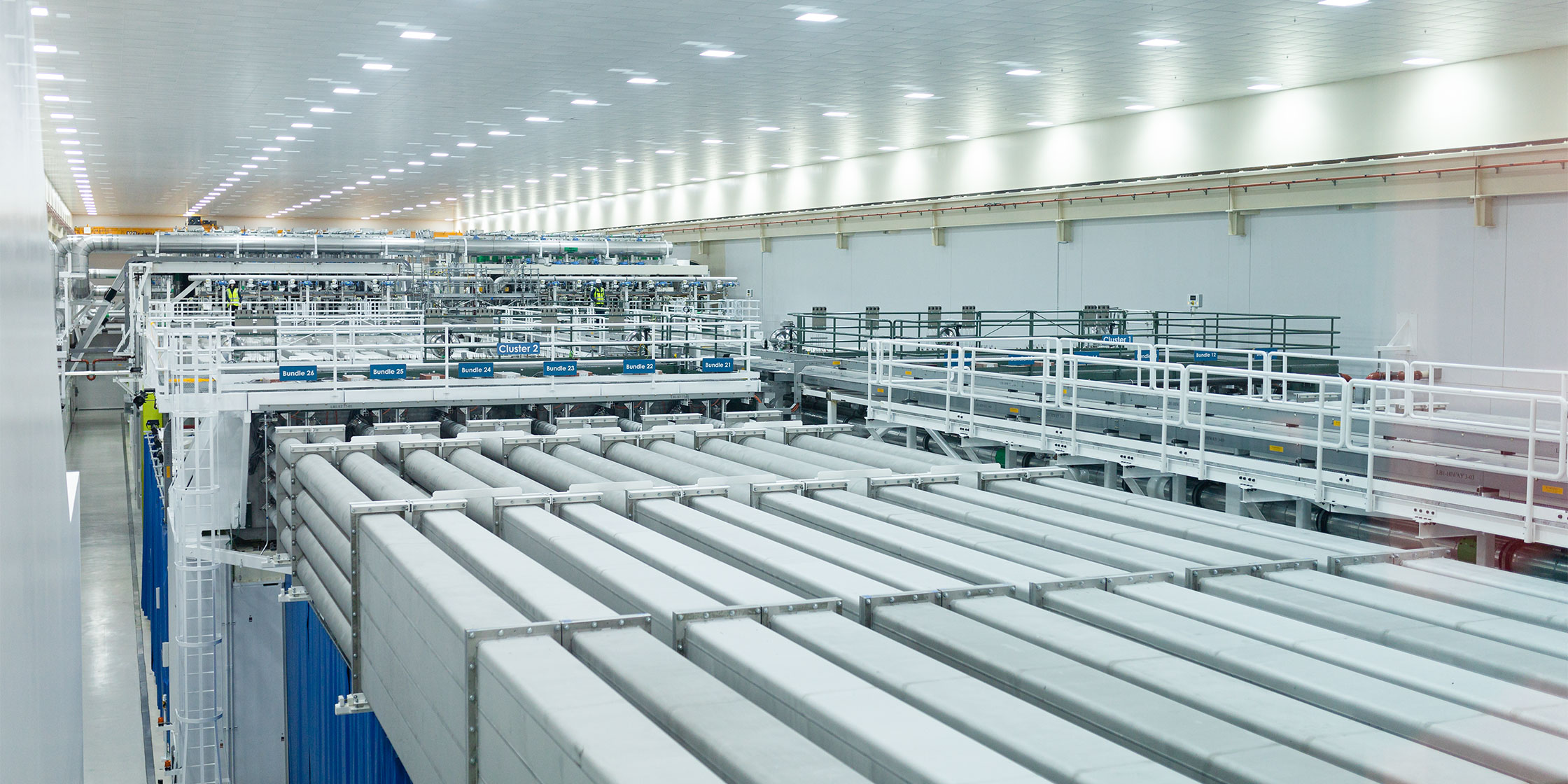
The laser bay room at the National Ignition Facility. The energy from each individual beam will be combined to create the world’s most powerful laser.
Constanza Hevia for Quanta Magazine
How do you do one of these experiments?
Basically, we take a tiny sample of material — like micron-size thickness — and we focus lasers on it. We tune the laser power as a function of time in a very accurate way that compresses the material in the way we want. If we just blast a shock wave through the material, we will melt it at relatively low pressure. For these planetary science applications, we don’t want to do that, because materials tend to be solid inside planets.
We use an instrument that is basically a small metallic box, where the sample is on the front. This box contains X-ray-sensitive detectors. When the material has reached the pressure we want to probe, we fire additional lasers that generate X-rays that will be transmitted through the sample, and then the detectors record the diffraction patterns.
What does it feel like walking around this type of facility?
The NIF building has shots 24 hours a day. Sometimes you have to go there in the middle of the night and walk this corridor, through a tunnel, and up a staircase to reach the conference room. It was certainly a little intimidating the first couple times I went there. Once you get to the conference room, it’s really nice. It almost looks like in the movies when they set up for the launch of a shuttle. You have all the people sitting at a desk, and everyone is assigned to a different aspect of the experiment: diagnostics, lasers and target. You have these huge screens, and then there’s the shot director, who controls everything.
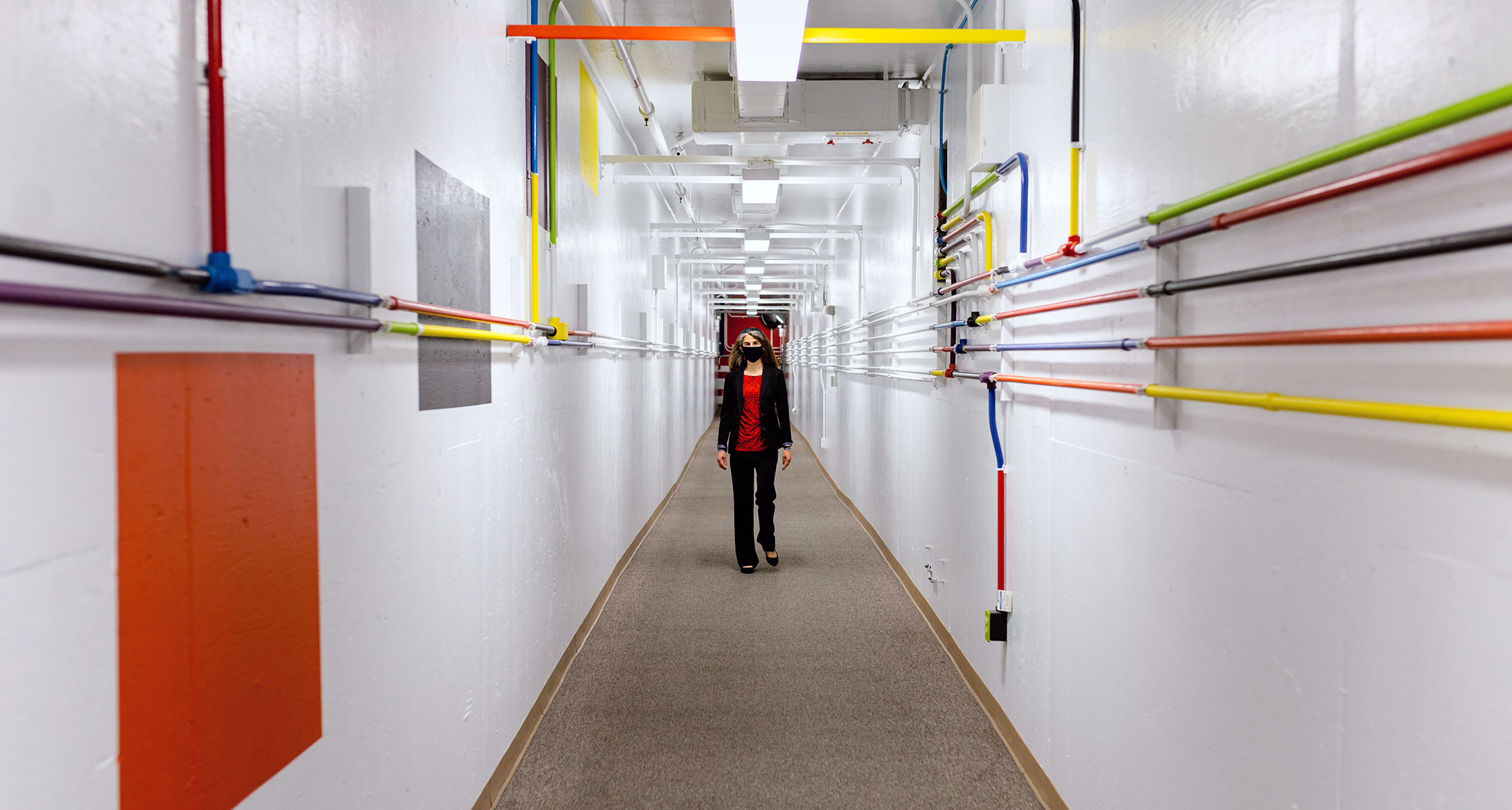
Coppari walks through the tunnel to the conference room at NIF.
Constanza Hevia for Quanta Magazine
What was the purpose behind your experiments on superionic ice?
There was this long-standing prediction of the existence of superionic water ice at extreme conditions, about two megabars, and thousands of degrees Kelvin. But experimentally it was very challenging to recreate those conditions in a laboratory. At first, I must confess I was skeptical whether we should try. But then I ran some calculations, and I looked at experiments I had done on another light material, magnesium oxide. I said if we do some improvements and increase the size of the sample by a factor of two, make it thicker, and maybe probe it a little longer, it could work. We could get a signal.
How did it feel when you succeeded? This was something people had been trying to prove since the 1980s, and suddenly you’re seeing it.
The funny thing is, as I was analyzing the data, I didn’t immediately realize what I was looking at. We had different experiments, and the pressures and temperatures we were probing were a little different. The diffraction signal seemed to be all over the place, and I scratched my head and said, “What does this mean? It shouldn’t go back and forth.” I had many versions of the analysis to make sure I wasn’t doing anything wrong. And then I was always getting the same result, and I thought, “OK, this is it.” It was exciting.
Did people in the field already know about this connection to ice giants?
The idea that superionic ice may be inside the ice giants was already put forward to explain some of the magnetic field features of the planets. There was a paper by a Harvard group who had done some modeling and predicted that, if the interior wasn’t just a fully fluid icy mantle but was stratified, then you could reproduce some of these magnetic features. Later, a German group argued that this stratification may come from water becoming superionic in the deeper layers of the planet. There was no experimental evidence for whether that was true or not. That motivated our study.
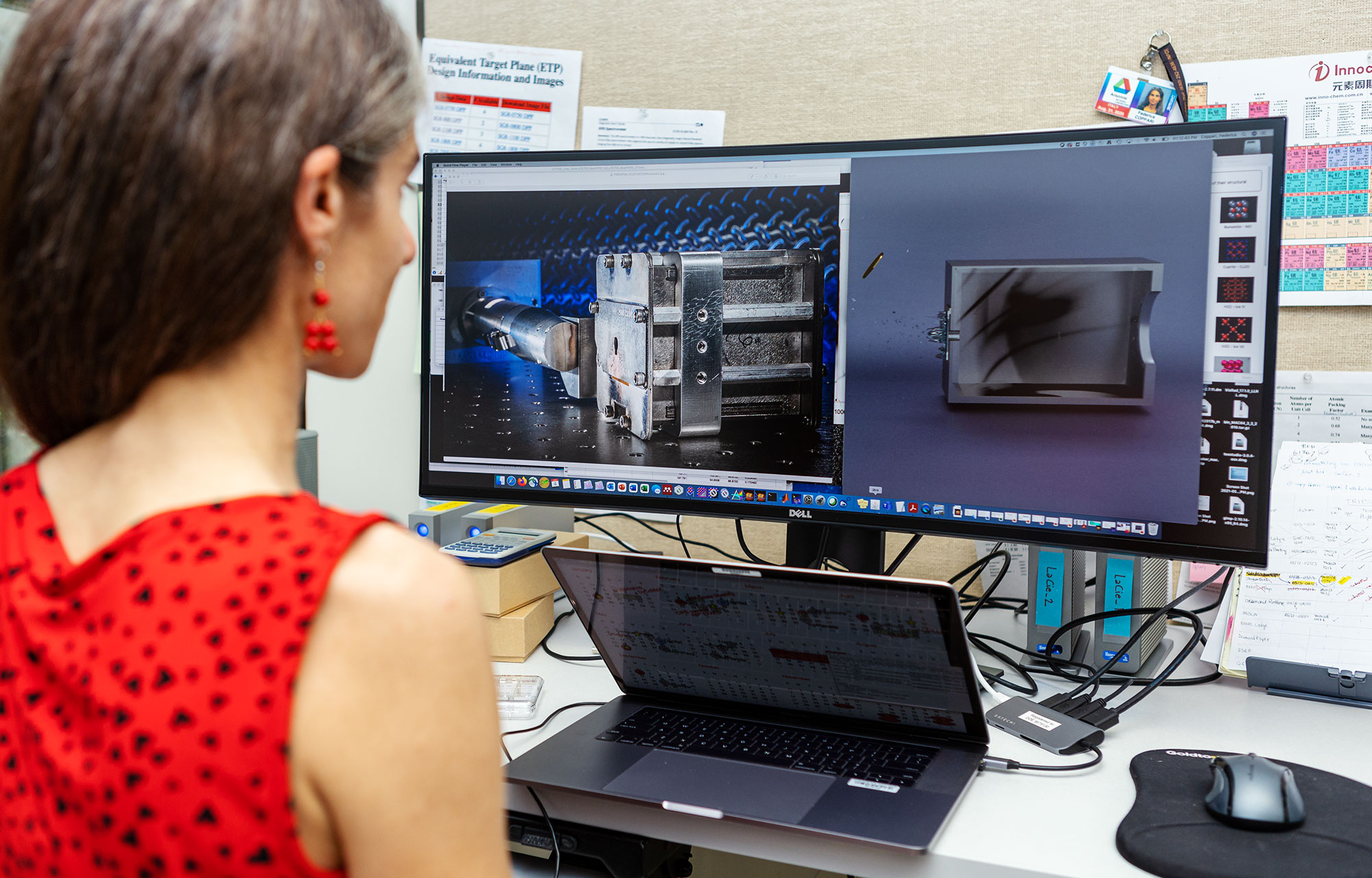
Coppari views some of the experimental apparatuses that will be used to collect data inside the experiment.
Constanza Hevia for Quanta Magazine
The more recent work on iron, how did that originate?
The motivation there is to understand what happens in the mantle of large extrasolar planets. We know the main components of the Earth’s mantle. We know that their convecting motions, together with a liquid-iron component of the core, generate the magnetosphere and tectonic plates, which are the basis for habitability.
Some extrasolar planets are expected to have a rocky interior structure similar to the Earth, so can they harbor life?
The first step is seeing what the materials do inside the mantle of the planet, where the pressures are higher than those inside the Earth. In the experiment, we saw that iron oxide undergoes a phase transition to a denser structure when we compress it. That phase transition doesn’t happen at the pressures existing in the Earth, so that’s already an important difference. The other difference is, if the planet is big enough, magnesium oxide — which is another component of the mantle — can undergo that phase transition.
Is this the best approach, to look at one component, then another component?
Our field is really just starting, so looking at one component material is easier than mixing the two together and seeing what happens. The next step is to mix them together.
What are some worries when doing an experiment like this?
One of the first experiments I was doing at Omega, I was really stressed out because it’s an expensive experiment, and in a shot day you get 14 experiments. You really need to make it count. I was stressed that I would set up the shot with a mistake, like the wrong energy, the wrong timing, the wrong diagnostic setup, because everything has to be timed super accurately. There are so many ways that you can fail an experiment, and one is making stupid mistakes. That was the worst fear I had.
Do you walk around and look down and think about what’s going on inside Earth?
I look up more than down. It’s incredible what I get a chance to do when I think about it. Like with any other work, there are tough moments, but it always makes me feel that I’m doing what I want. It’s a good motivation to move on and take the bad and the good.