For Embryo’s Cells, Size Can Determine Fate
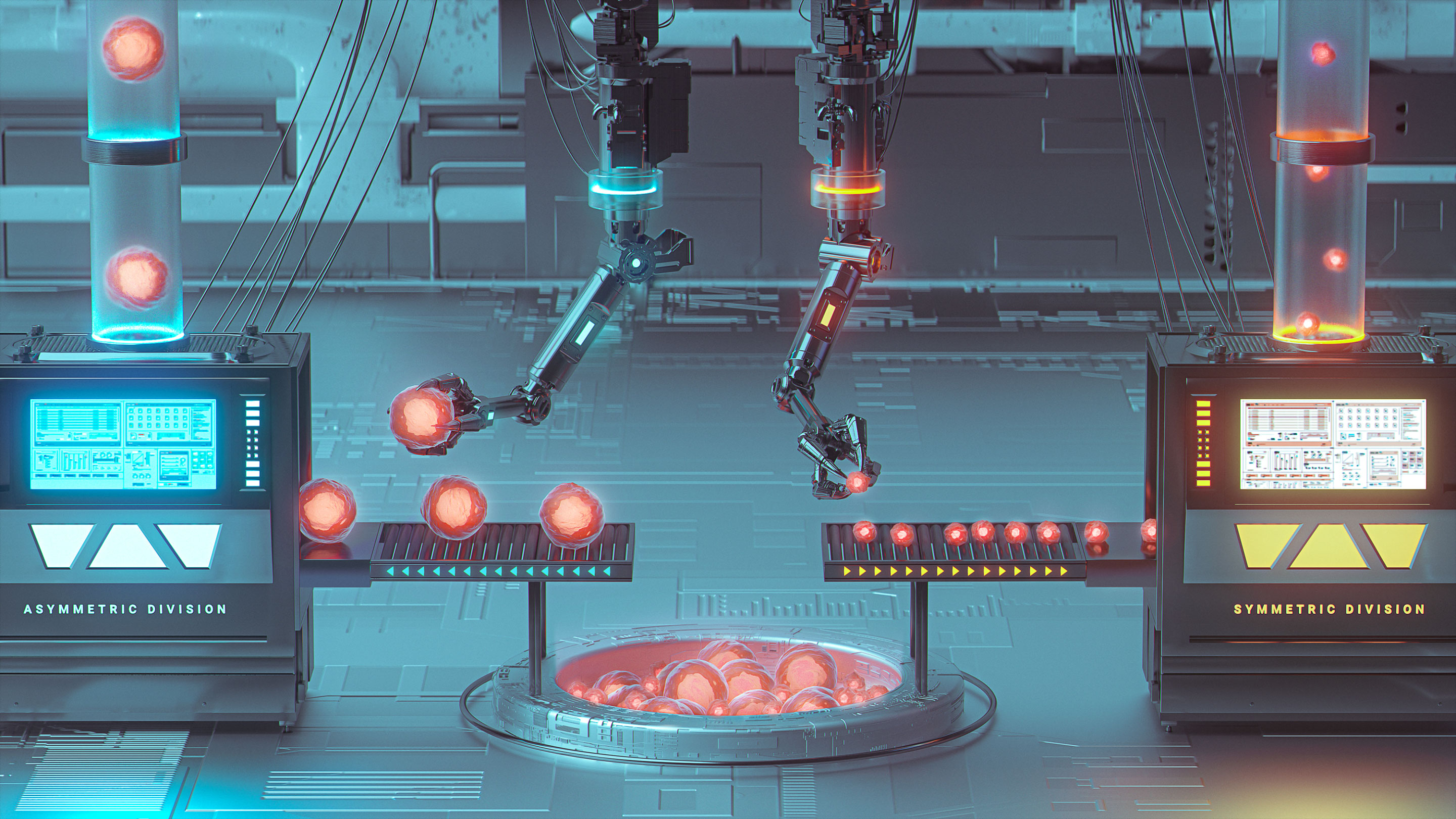
Early in embryonic development, it is common for cells to switch from dividing asymmetrically (into daughter cells with different sizes and developmental fates) to dividing symmetrically (into identical cells with the same fate). The size of the cells seems to be what determines when that switch occurs.
BakaArts for Quanta Magazine
Introduction
The developing embryo is a finely tuned machine. Its cells know what to do, and when to do it. They know to grow or shrink, to divide or lie dormant, to come together into a beating heart or hurtle through the bloodstream in search of a distant invader. And they know to do all that without a central command station or an objective map of their surroundings to guide them.
Instead, cells are left to devise their own strategies for calculating precisely where to go and what to become. Those calculations depend on a veritable cocktail of signals, some of which have long been established as obviously important — chemical and electrical gradients, the activity of gene networks, patterns of overlap between spreading fields of molecules.
But recently experts have also started to pay attention to another, often overlooked set of factors: physical constraints such as size. In new work published today in Nature Physics, a team of researchers reported that during the early development of the roundworm Caenorhabditis elegans, a mechanism based on the size of embryonic cells helps to determine the type of mature tissues they will eventually produce.
While examining the biochemical process that triggers cells to divide either asymmetrically or symmetrically, the scientists discovered that size was the ruling element — namely, that the size of the cells dictated the pattern that led to one kind of division or another, and ultimately to one kind of lineage or another. “The biology is actually exploiting this fact … to generate a set of outcomes that are required for the development of the organism,” said Martin Howard, a computational biologist at the John Innes Center in England who did not participate in the study. In this case, cells used innate constraints on their size to specify the lineage that would later give rise to the worm’s sex cells. But more broadly, the findings also point to the possibility of a role for physical cues in the behavior of stem cells and the operation of other developmental systems.
Broken Symmetries
Just as the universe was born from the breaking of symmetry, so are each of the animals and plants that inhabit the Earth. During early embryonic development, cells undergo at least one asymmetric division and sometimes several more: They produce daughter cells that differ in size and fate, laying the foundation for the later specification of various distinct cell types. To cement these budding lineages, and to stop creating new ones, the cells then have to shift gears and start dividing symmetrically.
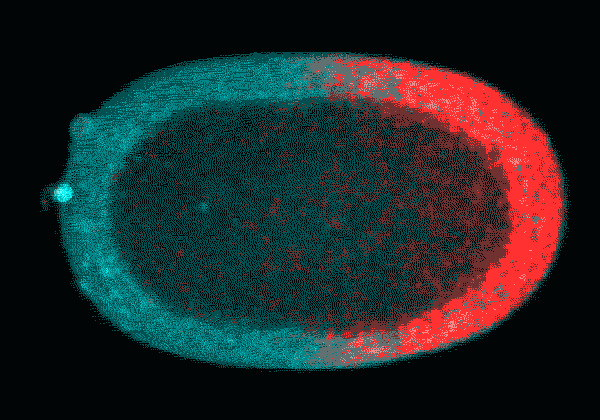
In this time-lapse video of an early C. elegans embryo, the first rounds of cell division are asymmetric: One daughter cell is larger than the other. The P cell lineage that eventually gives rise to germ cells appears red. With each round of division, the cells of the P lineage get smaller until they are too small to divide unevenly anymore.
Courtesy Nate Goehring
For instance, when the worm embryo is still a single cell, proteins on its outer membrane create two uneven, yin-and-yang-like domains that tell the cell where to split. That system for designating asymmetric cell division is called polarity. One lineage of embryonic cells in C. elegans (known as the P lineage) uses polarity to divide asymmetrically four times; the fifth division is then symmetric, permanently establishing the germline responsible for egg and sperm cells.
This polarization system resembles the Turing-pattern model for the mechanisms that may guide the formation of spots or stripes. A spot may form on a leopard, for example, because one activator molecule diffuses through skin tissue and stimulates the production of pigment while an inhibitor molecule suppresses pigmentation in the surrounding region. The size and distribution of the spots therefore depends on kinetic factors such as how quickly each of the molecules diffuses.
That’s what happens with polarity, too. Two proteins that exclude each other activate on the cell membrane at opposite ends of the cell, so that where one is present, the other can’t diffuse. They move at different rates, and at the border between their two asymmetric domains, the cell divides. One daughter remains part of the P lineage, while the other is destined for another fate. As with Turing patterns, the system works because it strikes a careful balance between its size and how quickly the proteins spread.
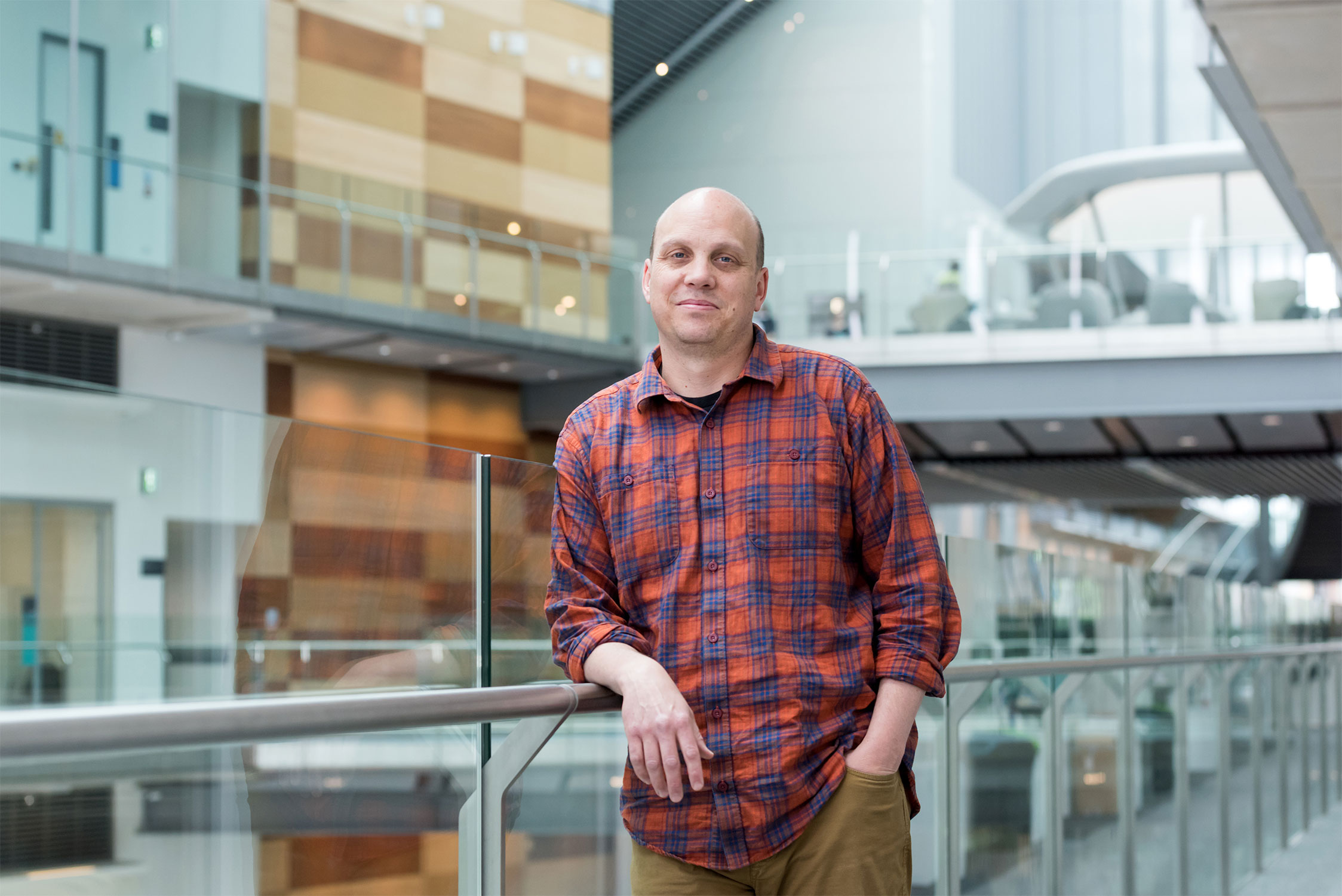
Working with computer models, Nathan Goehring, a molecular biologist at the Francis Crick Institute, realized that the size at which cells became too small to polarize corresponded to the size at which cells in C. elegans committed to becoming sex cells.
Courtesy Nate Goehring
Nathan Goehring, a molecular biologist at the Francis Crick Institute in London, wanted to probe that balance. He and his colleagues played around with previously published polarization models, testing what would happen when they made their theoretical cells larger or smaller. Their simulations indicated that if a cell got too big, more than two protein domains would emerge, leading to loss of polarity.
A more interesting result was that when the cell got too small, only one domain dominated, uniformly diffusing across the membrane. Again, polarity broke down, this time leaving symmetric division as the only option. The threshold cell circumference at which that happened hovered around 41 microns.
To Goehring, that figure looked familiar. In an early C. elegans embryo, cells don’t grow between divisions, meaning that as they divide, they get smaller and smaller. And 41 microns was strikingly similar to the size of the last cells to divide asymmetrically in the P lineage. Could it be that the daughters of those cells were simply too small to polarize — that size was the determining factor in the switch to symmetric division and the designation of their fate?
Scales and Rulers
To find out, the scientists measured the kinetic properties of an important polarizing protein in normal C. elegans embryos and in embryos whose sizes they had genetically manipulated. As expected, the protein’s diffusion rate and other qualities did not change, even when the cells got bigger or smaller. Instead, the patterning system had its own intrinsic scale, one that didn’t adjust to the overall size of the cell.
By controlling the sizes of the initial embryos, the team was then able to show that there was a minimum size threshold for the P lineage cells, below which they could not set up the polarization pattern. Those smaller cells lost the ability to polarize after just three cell divisions, not four. “Just by manipulating the size of the embryo, we’ve taken a cell that normally would be able to polarize and divide asymmetrically and turned it into a cell that doesn’t polarize and divides symmetrically,” Goehring said.
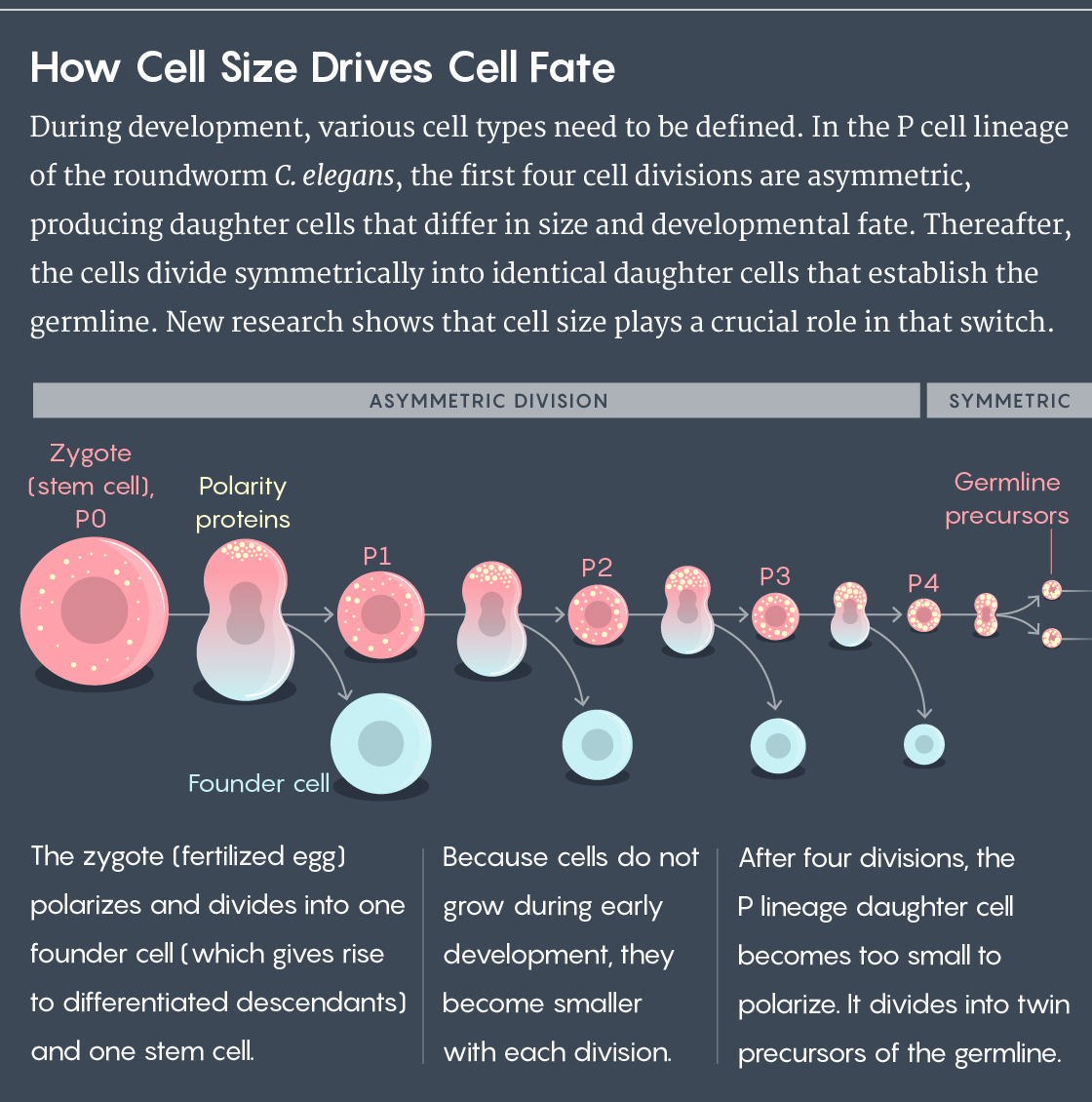
Lucy Reading-Ikkanda/Quanta Magazine
Moreover, a perusal of previous research revealed that two other worm species have one extra asymmetric division in their P lineage. Their P lineage cells tend to start bigger (and stay bigger) than those of the early C. elegans embryo, in keeping with Goehring’s theory. Whether the same mechanism is truly at work in those species remains to be tested, but it doesn’t seem like a coincidence.
Cells have seemingly evolved to take advantage of the intrinsic limitations of their patterning process — using it as a ruler of sorts — to determine whether to become germ cells. “The specification [of the germ cells] is a kind of self-organized property of the patterning system,” Howard said.
And that’s a “genuinely interesting” way to think about the system, said Timothy Saunders, a biophysicist at the Mechanobiology Institute of the National University of Singapore who was not involved in the study. “This idea, that by just simply making things smaller you can naturally switch the type of division, is very neat.”
A New Perspective
These findings come at a time when scientists are widening their view of what controls biological systems to encompass more than genetics alone. “The gene does not exist in a vacuum,” Saunders said. “And we’re realizing more and more that the mechanical environment in which those genes are operating matters” — including for decisions about cell fate.
Researchers have found, for instance, that cancer cells respond to the stiffness of the surrounding tissue and other environmental factors. Stem cells, when subjected to certain physical forces, can also be induced to change their behavior and fate. And self-organizing models of tissues called organoids can’t grow properly on a flat dish. “I think our data nicely fits into that idea, that the physical environment matters, that the cells are measuring these things,” Goehring said. “In this case … the cells are sensing size.”
While this work focused on a particular cell lineage at a particular stage of development in a particular species, “it’s something that could have more general resonance,” Howard said. The polarity network in the P lineage is strongly conserved among animal species, including in humans. But because mammalian cells lack a “nice clean topology,” this will be particularly challenging to study, according to Saunders — though he expects it to still be relevant in those systems.
Other processes that involve cells getting smaller with each division might also be using size to make decisions about fate. Neural stem cells in flies and certain plant cells, for instance, shrink with each division until they stop dividing altogether. “While we don’t know that there’s a strict size sensor in any of those systems,” Goehring said, “it’s sort of consistent with this idea that there may be size-dependent switches in fate.”
The same goes for stem cells in the mammalian gut, which divide rapidly in spatially constrained crypts. They need to choose when to divide asymmetrically (into one stem cell and one specialized cell) or symmetrically (into either two stem cells or two specialized cells). That choice is critical for maintaining stem cell populations in the organism — and it’s not always clear how it’s getting made.
Perhaps cell size will once more turn out to play a role. “I think that idea is something that’s going to be universal,” Goehring said.
There are already hints of that. Cells seem to build specific numbers of organelles, in specific proportions, by using their cytoplasm as a limiting pool of gradually depleted building blocks. And in a paper published in Developmental Cell in June, a team of researchers proposed a model for how the embryo’s genome gets activated after fertilization. According to their work, that happens only after cells reach a certain size threshold: As the early frog embryo divides, its cells get smaller, and it has less cytoplasm relative to its DNA. As the concentration of a particular type of DNA-condensing protein decreases, it frees up more and more of the genome to be expressed, until finally transcription gets turned on.
Of course, in all this work, questions remain, particularly about how the systems stay resilient to natural variations in cell size, and how size might affect differentiation much later in development. Still, Goehring said, it’s now crucial to turn to processes “that maybe we haven’t looked at because we haven’t been thinking about size.”
“We need these sorts of theoretical ideas in order to unlock how [cell fate decision-making] is working,” Howard said.