How a Biofilm’s Strange Shape Emerges From Cellular Geometry
Introduction
Biofilms lead lives of liminality. Just a few cells thick, these layered communities of microbes anchor themselves to solid surfaces at interfaces — where rocks meet salt water in tide pools, between plants and dirt in root systems, or on the saliva-covered surface of your teeth. Amalgamations of single cells, biofilms grow and develop into unified life forms that can split back into their component cells under duress. Biofilms, then, are somehow both unicellular and multicellular — and simultaneously neither.
Biofilms have emergent properties: traits that appear only when a system of individual items interacts. It was this emergence that attracted the biophysicist Peter Yunker to the microbial structures. Trained in soft matter physics — the study of materials that can be structurally altered — he is interested in understanding how the interactions between individual bacteria result in the higher-order structure of a biofilm.
As cells divide and a biofilm grows, it doesn’t simply expand outward. What starts as a flat, smooth layer of cells stretches and pulses. Strange, sticky shapes appear as the bacteria reassemble into ridges and depressions that warp and buckle, almost as if the collective is breathing. In recent years, researchers have been studying the role of shape and geometry in biofilms and how physical laws, such as those governing cellular metabolism and the diffusion of nutrients, determine how biofilms grow and thrive.
“If we only look at the growth rate of cells,” Yunker said, “then we’re missing an equally important component, which is how the emergent-group geometry impacts a biofilm.”
Recently, in his lab at the Georgia Institute of Technology, Yunker and his team created detailed topographical maps of the three-dimensional surface of a growing biofilm. These measurements allowed them to study how a biofilm’s shape emerges from millions of infinitesimal interactions among component bacteria and their environment. In 2024 in Nature Physics, they described the biophysical laws that control the complex aggregation of bacterial cells.
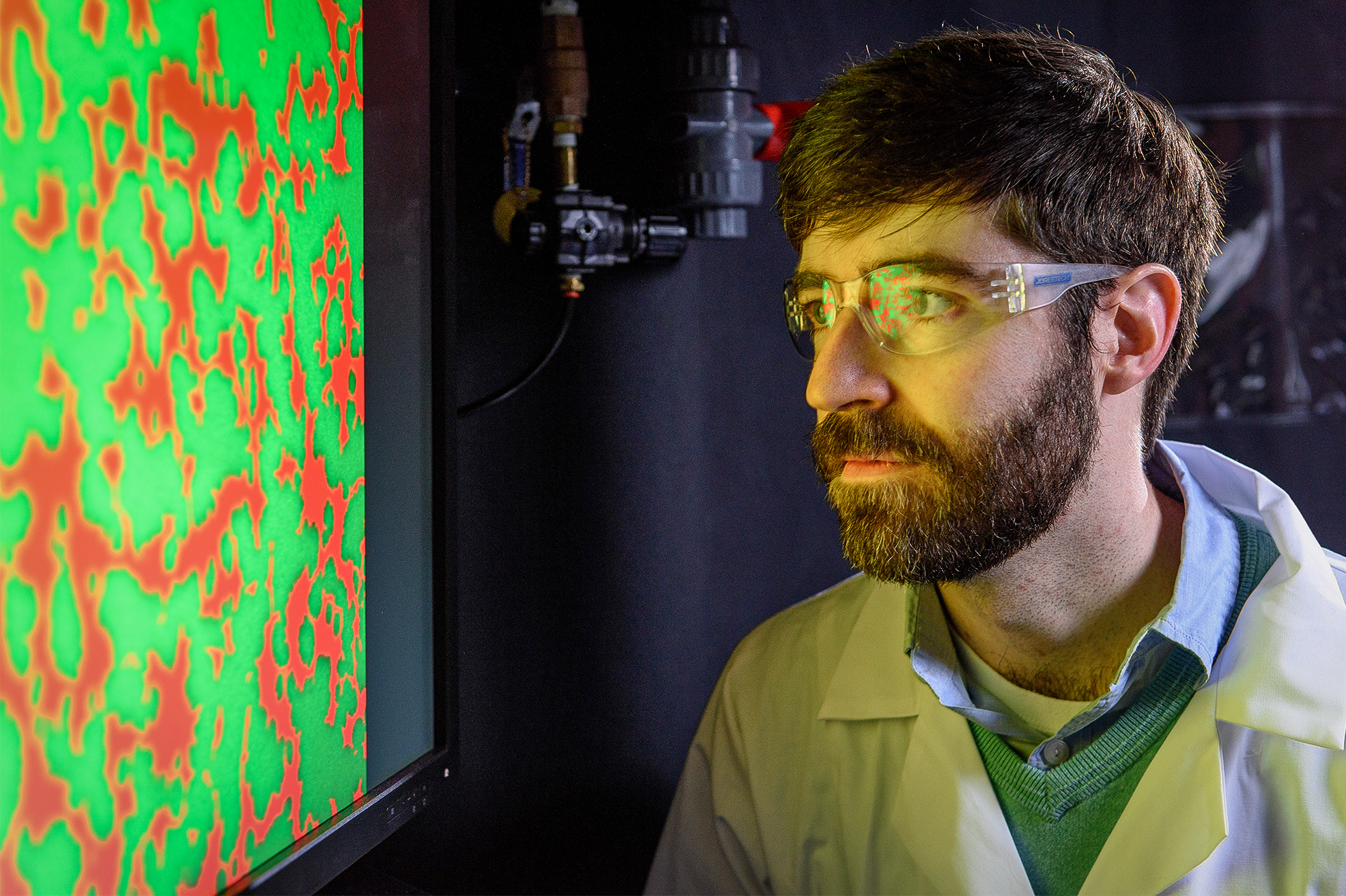
At the Georgia Institute of Technology, Peter Yunker treats the microbial communities known as biofilms like the colloids he studied as a physicist. In both, physical interactions between particles can produce “very interesting collective phenomena,” he said.
Ben Brumfeld
The work is important, Yunker said, not only because it can help explain the staggering diversity of one of the planet’s most common life forms, but also because it may evoke life’s first, hesitant steps toward multicellularity. Somehow, many times over the history of life on Earth, single cells that previously experienced life as a solo endeavor evolved to work cooperatively as a collective — another example of emergence.
“The characteristics of multicellularity are very strongly influenced by physics,” said Stuart Newman, a biophysical developmental biologist at the New York Medical College.
Scientists are inching ever closer to figuring out the biophysical rules that govern how and why those first cells clumped up and stuck together. These studies provide clues about the geometric principles that can generate dazzling morphologies, said Omaya Dudin, an evolutionary cell biologist at the University of Geneva.
“We cannot understand the cell only by looking at its genomics,” he said. “We need to understand what it looks like.”
Metabolic Topography
It isn’t existential loneliness that drives microbes to form biofilms; it’s the benefits of communal living. Microbes that can form such a mass — and many can, as biofilms are one of the planet’s most common life forms, encompassing 40% to 80% of all prokaryotic life — gained the ability to anchor themselves to stationary surfaces, such as the grooves of our teeth or the bumpy exterior of a boulder. A sticky, sugary, mucus-like extracellular matrix physically binds biofilm members to each other and to the solid substrate upon which they grow.
Large clumps of microbes can digest more nutrients, making that energy more widely available. They are also less likely to become dinner themselves, since their neighbors provide physical protection. Agglomerates of cells are shielded from everything from harmful chemicals to ultraviolet light to desiccation. They become something between a community and multicellular life.
“Cells have the capacity to cooperate, to socialize,” said the evolutionary biologist Iñaki Ruiz Trillo of the Catalan Institution for Research and Advanced Studies in Barcelona. “It’s intrinsic to cellular ecology.”
But group living also comes with drawbacks. As more and more cells cluster together, it becomes harder for oxygen and nutrients to diffuse to all cells. The outermost microbes, with the most access to the external environment, gobble up nutrients to fuel their own growth, leaving internal cells starved.
It doesn’t take a huge number of cells for resource distribution to become a problem. Research by the microbiologist Lars Dietrich of Columbia University has shown that in biofilms just 50 micrometers deep — the equivalent of about 20 to 50 cells — oxygen levels plummet in the interior. This means that the physical structure of a biofilm is vital to the group’s collective health.
“On some level, all biological shape and form is ultimately constrained by what’s metabolically feasible,” Dietrich said. “Each structure needs to get access to the nutrients, oxygen or whatever else they need.”
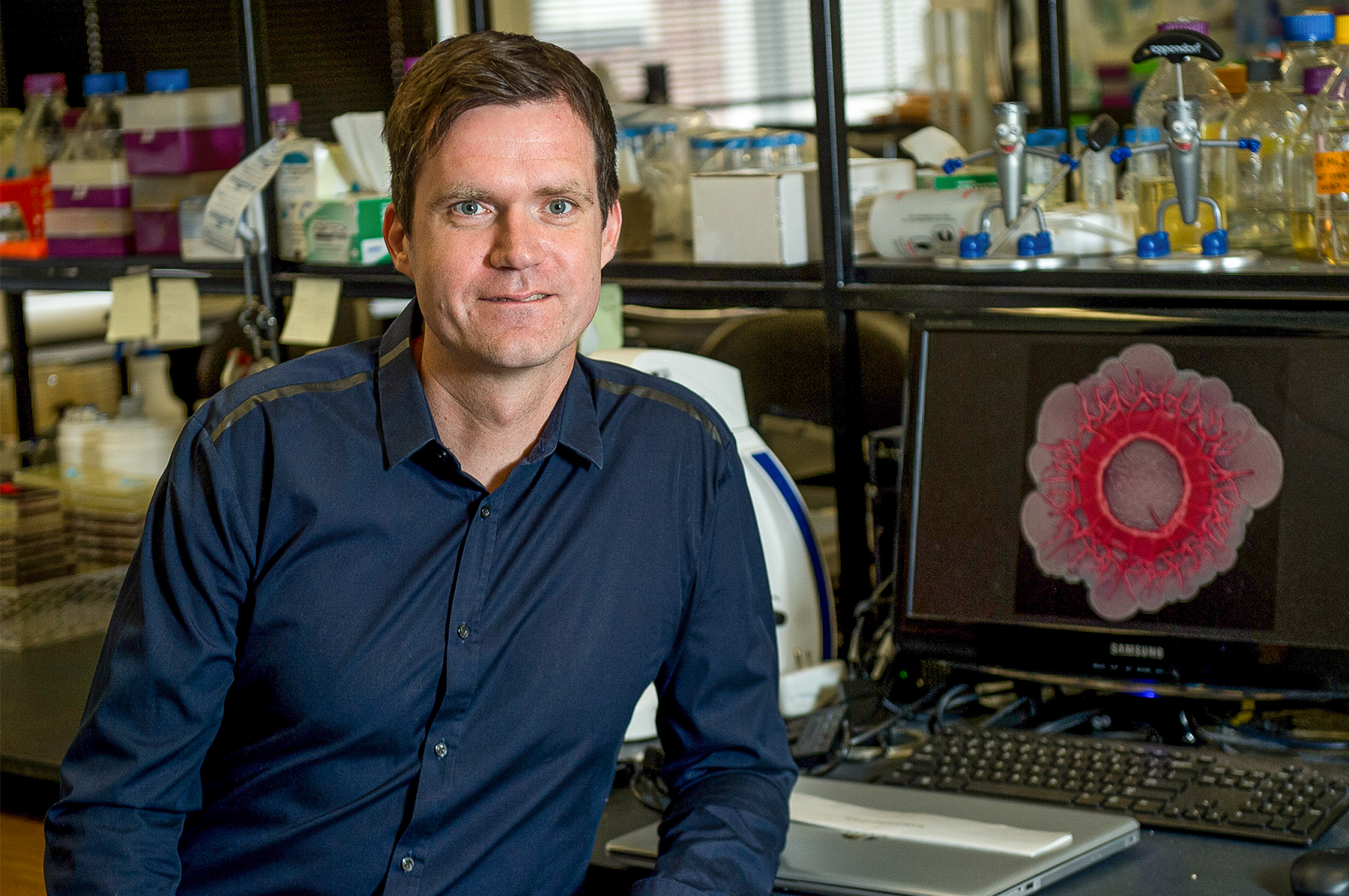
The microbiologist Lars Dietrich of Columbia University has found that biofilms only 20 to 50 cells thick can have resource distribution problems.
Diane Bondareff Photography
His experiment started with a small colony of Pseudomonas aeruginosa bacteria growing on an agar plate in his lab — a small, whitish dot, roughly the size of the period at the end of this sentence. The dot grew larger as the days passed. Then, in an eyeblink, magic happened.
Instead of continuing to grow into an ever-larger circle, the bacterial colony shape-shifted. The fondant-smooth top began to wrinkle and crumple. Soon, the nascent biofilm had acquired the origami-like folds of the neocortex. When Dietrich changed the nutrients in the growth medium — providing a different sugar source, less protein or more salt — an entirely different topography emerged.
In all the scenarios Dietrich tested, the resulting biofilm looked nothing like the single cells or small colony from which it arose. But when he zoomed in with his scanning electron microscope, he was able to see the internal scaffolding structures made up of billions of individual Pseudomonas. He realized the close connection between the biofilm’s structure and its metabolism.
This realization prompted him to take a deep dive into biofilm microanatomy. He and his team focused on striations, vertical sections of densely packed bacteria. The cells at the top of the striations had ample oxygen; those at the bottom had virtually none. His lab found that the cells along this gradient could sense the varying levels of oxygen, which triggered a series of biochemical alterations. Gene expression changed. So did the makeup of the biofilm’s sticky extracellular matrix and the physical arrangement of its cells. Dietrich and colleagues published their results in PLOS Biology in 2024.
Biofilm shape, then, alters nutrient availability, and nutrient availability alters metabolism and cell survival. “By using different types of metabolism along these gradients, [a biofilm] can make sure that everybody survives,” Dietrich said.
Determining how structure impacts nutrient availability is important, Yunker said. But he wanted to take the study of biofilm geometry further to understand how interactions between cells can create the strange shapes in the first place.
Microbial Physics
With his background in soft matter physics, Yunker thinks about the emergence of a biofilm’s wrinkles through a different lens. The biofilms he watched under the microscope reminded him of substances called colloids, which he studied in his days as a doctoral student. Colloids are created when small particles are dispersed in another material — for example, gelatin is a colloidal solid, mayonnaise is a colloidal liquid and fog is a colloidal gas. The particles making up the colloids Yunker had investigated were about the same size as bacteria. As in biofilms, various physical properties of colloids emerge from simple interactions between neighboring particles.
“[Colloids] can exhibit very complex, very interesting collective phenomena just from their physical interactions,” Yunker said. “One particle pushes on another particle, which pushes other particles, leading to this cascade of interactions that then produces a full-scale phenomenon.”
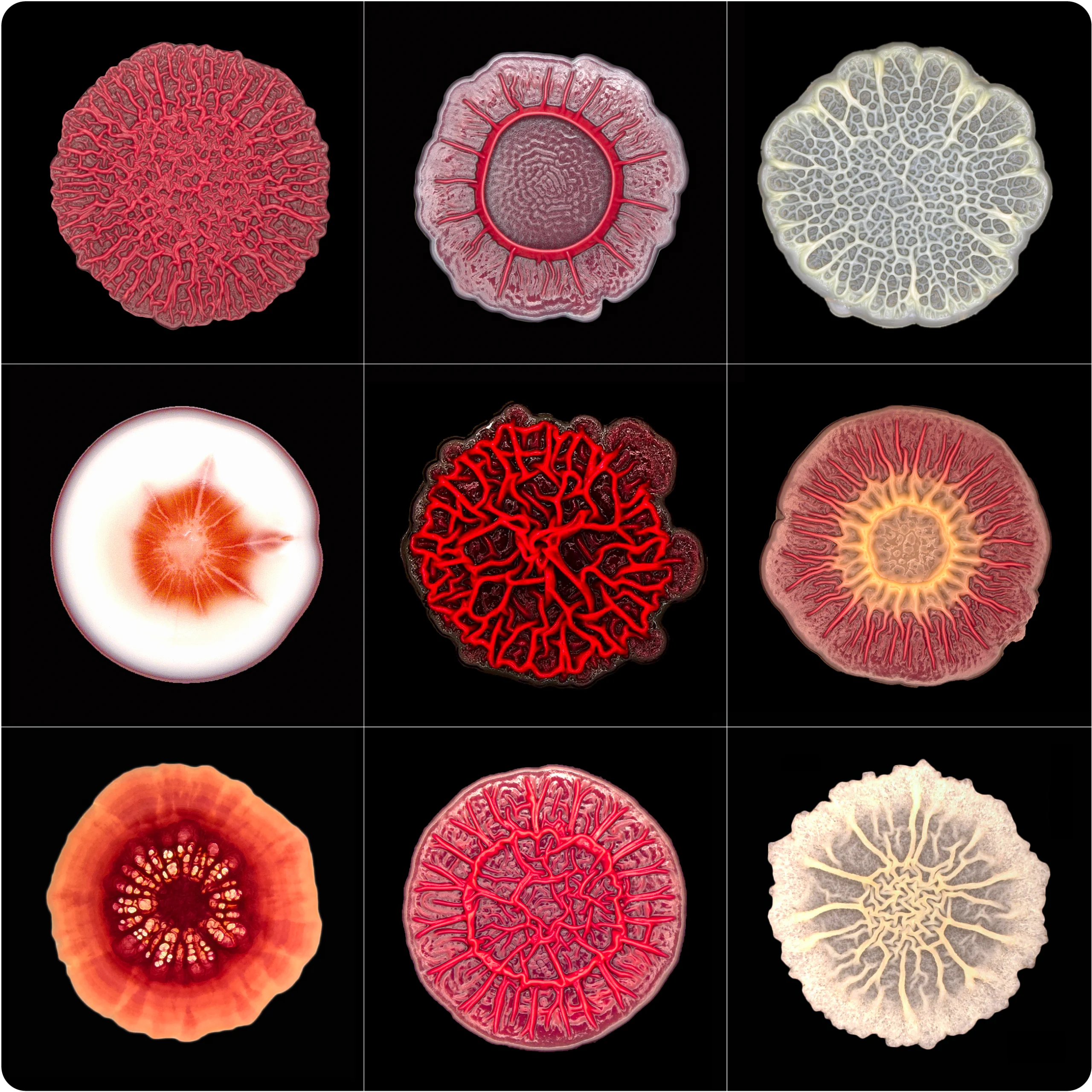
Biofilms can form many unique shapes. When they’re given different nutrients — a new sugar source, less protein or more salt — entirely different topographies emerge.
Courtesy of Lars Dietrich
In colloids as in biofilms, these interactions are governed by two opposing forces. One is repulsive: Two particles or two cells can’t physically occupy the same space at the same time. The other force is attractive. Cells are covered with sticky proteins that can fasten two cells together, much like the medium binding a colloid. If the repulsive force is stronger, cells don’t aggregate. But if the attractive force is stronger, it can spark the initial formation of a biofilm.
What differentiates colloids from biofilms is growth, which a biofilm must balance between the horizontal and the vertical. It’s not unlike urban sprawl. Both Houston, Texas, and Queens, New York, house populations of around 2.3 million people, but they have completely different urban geometry. Around Houston, cheap and abundant land lets residents spread out, with mostly horizontal growth. Queens, on the other hand, is hemmed in by water and surrounding municipalities, so residents build more vertically. That’s how 2.3 million residents occupy 640 square miles in Houston and 109 square miles in Queens.
Just like Texans and New Yorkers, biofilms weigh the trade-offs of horizonal versus vertical growth. “A single cell can only be in one place at any moment in time,” Yunker said. “The more that cells at the edge of a biofilm grow up, the less they can grow out.” This results in a kind of cellular geometry, which Yunker found plays an outsize role in a biofilm’s overall fitness, measured by its ability to expand and take in nutrients.
“When we look at an animal, geometry is the first thing that comes to mind,” said Ming Guo, a mechanical engineer and biophysicist at the Massachusetts Institute of Technology who was not involved in the research. “Underneath that is the detail — the genetics, epigenetics, protein profile and cell types — that together define the entire biology.”
Yunker decided to focus on what happens at a biofilm’s leading edge — the outer perimeter where cells most actively grow, divide and shape the emerging structure. To measure growth there, Yunker used white light interferometry, a technique that uses the interference patterns of light waves to measure physical characteristics with nanometer resolution.
Using a biofilm of Vibrio cholerae bacteria, Yunker’s team described the shape of the emerging biofilm as a spherical cap that resembles a contact lens. Eventually the cap grows too thick for nutrients to diffuse upward from the agar plate to the cells at the top of the cap and for oxygen to diffuse downward from the air to the cells at the bottom.
A truly solo cell in this situation would simply move elsewhere. However, the cells smack in the middle of the growing biofilm are glued in place by an extracellular matrix. Too far from food to obtain energy and too far from fresh air to receive oxygen, these cells are stuck. Lacking nutrients, they divide more slowly — not nearly fast enough to maintain the shape of the spherical cap, which begins to deform.
While the middle section collapses, the expanding outer perimeter of the biofilm does not. This leading edge preserves the shape it had when it was part of the spherical cap, and a brand-new shape emerges across the entire collective.
No matter how large a biofilm got, Yunker found, the geometry at the outside edge remained constant. This consistent geometry emerged as a consequence of the cells’ interactions with each other and their environment. “It all comes down to just the shape of the pile of cells at the edge,” he said. “That’s what matters most.”
Yunker and his team went further and measured the contact angle between the expanding lip of the biofilm and its substrate — a function of the number of cells at the edge and how “sticky” those cells are. The stickiness of the biofilm’s cell matrix is determined by a range of factors, such as its chemical composition and the size and shape of the component microbes.
A trade-off between vertical and horizontal growth emerged. Tackier cells with a higher contact angle showed increased vertical growth and a reduced ability to spread horizontally. Less gluey cells, on the other hand, had a lower contact angle and spread more readily across a surface.
The researchers found that the perimeter’s contact angle is the most important factor in a biofilm’s overall growth and fitness. It determines the availability of nutrients, the rate at which cells divide, and the rate at which bacteria die off. In turn, the combination of these three factors ultimately controls the complex architecture of the emergent biofilm.
“We think that this is likely a very common occurrence in nature, where, many times, when you have simple cellular collectives, just through the very fact that they are interacting, it will lead to emergent physical traits,” Yunker said. “These will very often have biological consequences.”
Yunker’s work with the contact angle is a key example of how local factors can have macro consequences for life, said Guo, who studies the geometry of developing embryos. The results have implications for his own lab, he said, and for our understanding of how a group of individual cells becomes one multicellular individual.
“This is going to be really interesting for us to further dive down and understand the rules that regulate how cells talk to their neighbors,” he said. He hopes researchers can build on the work to show how these local communications lead to the emergence of a global shape. With enough information, Guo said that he might one day be able to predict an organism’s final form based on these cell-to-cell interactions.
“That’s the dream,” he said. “We hope to have a big, unified model.”