It Might Be Possible to Detect Gravitons After All
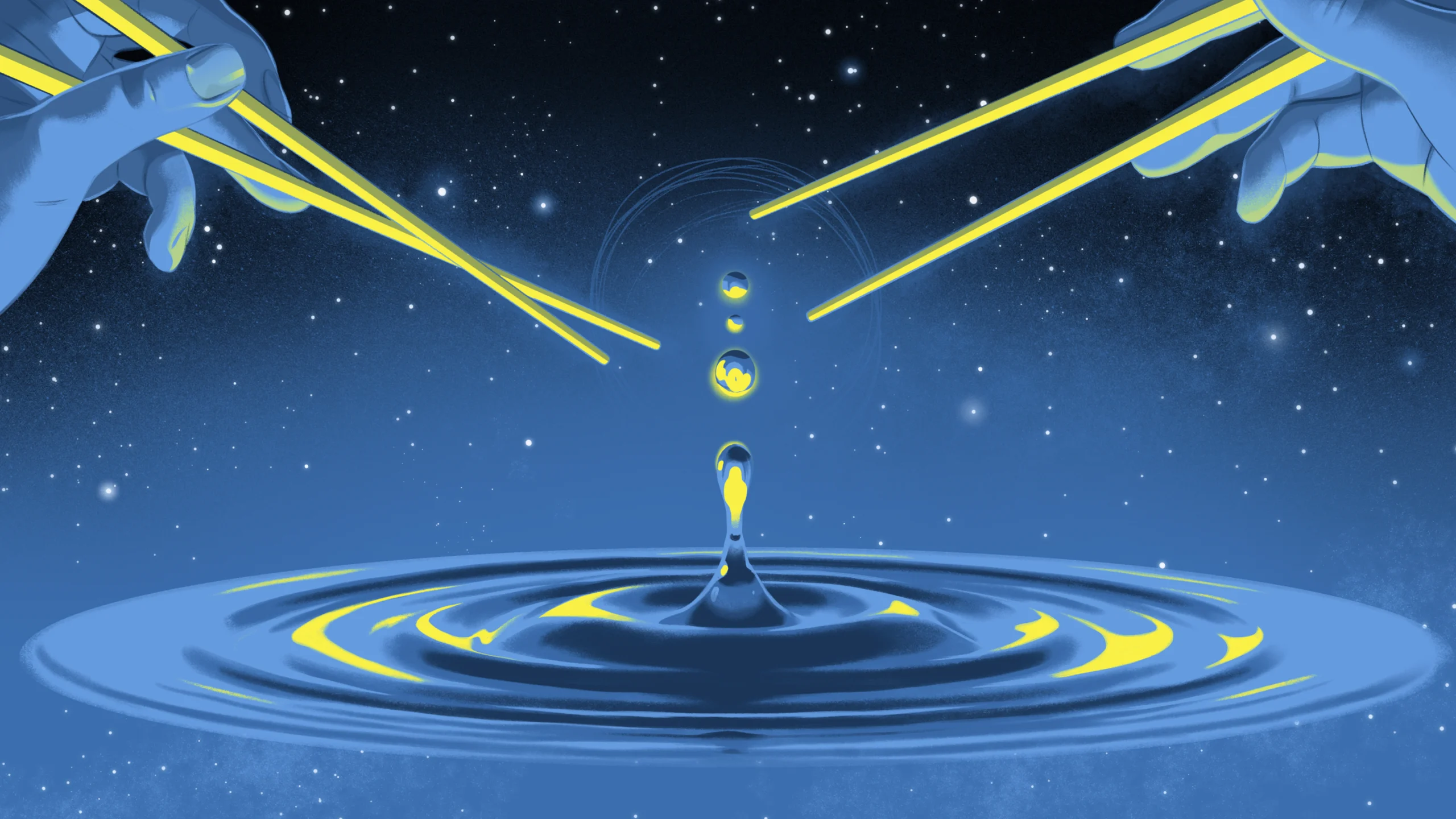
Capturing a graviton would be akin to noticing just one molecule in an ocean wave.
Señor Salme for Quanta Magazine
Introduction
Detecting a graviton — the hypothetical particle thought to carry the force of gravity — is the ultimate physics experiment. Conventional wisdom, however, says it can’t be done. According to one infamous estimate, an Earth-size apparatus orbiting the sun might pick up one graviton every billion years. To snag one in a decade, another calculation has suggested, you’d have to park a Jupiter-size machine next to a neutron star. In short: not going to happen.
A new proposal overturns the conventional wisdom. Blending a modern understanding of ripples in space-time known as gravitational waves with developments in quantum technology, a group of physicists has devised a new way of detecting a graviton — or at least a quantum event closely associated with a graviton. The experiment would still be a herculean undertaking, but it could fit into the space of a modest laboratory and the span of a career.
“It’s something that can be reached in a few years of research,” said Matteo Fadel, an experimentalist at the Swiss Federal Institute of Technology Zurich (ETH Zurich) who was not involved in the proposal.
“It’s a very original proposal and well thought-out,” said Frank Wilczek, a Nobel Prize-winning physicist at the Massachusetts Institute of Technology with a long-running interest in graviton detection. “It would be real progress in the field.”
Currently, Albert Einstein’s general theory of relativity attributes gravity to smooth curves in the space-time fabric. But a conclusive graviton detection would prove that gravity comes in the form of quantum particles, just like electromagnetism and the other fundamental forces. Most physicists believe that gravity does have a quantum side, and they’ve spent the better part of a century striving to determine its quantum rules. Nabbing a graviton would confirm that they’re on the right track.
But even if the experiment is relatively straightforward, the interpretation of what, exactly, a detection would prove is not. The simplest explanation of a positive result would be the existence of gravitons. But physicists have already found ways to interpret such a result without reference to gravitons at all.
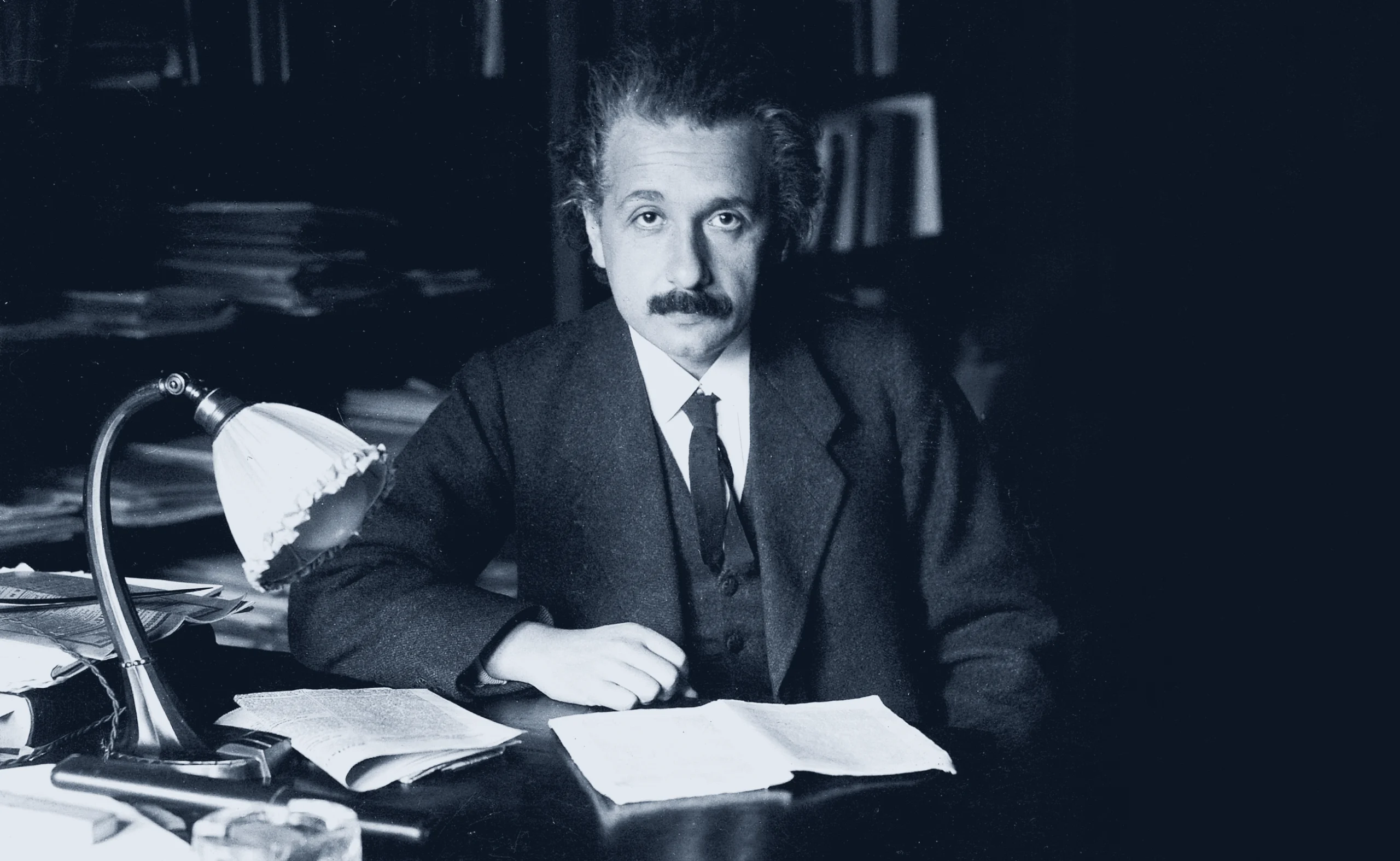
Albert Einstein published the current theory of gravity, called general relativity, in 1915, a few years before this photo was taken in his office at the University of Berlin.
ETH Zurich University Archive
The discussion recalls a messy, largely forgotten episode from the dawn of the quantum era. In 1905, Einstein interpreted experimental data to mean that light is “quantized,” coming in discrete particles now called photons. Others, including Niels Bohr and Max Planck, thought that the classical, wave nature of light might still be saved. It would take seven decades for physicists to undeniably establish that light is quantized, largely because of the subtle nature of quantumness.
Most physicists presume that everything in the world is quantized, including gravity. But proving that assumption will entail a new war, one that has only just begun.
Clicks From Gravity
It’s hard to experimentally probe gravity because the force is extremely weak. You need huge masses — think planets — to significantly warp space-time and generate obvious gravitational attraction. By way of comparison, a credit card-size magnet will stick to your fridge. Electromagnetism is not a subtle force.
One way to study these forces is to disturb an object, then observe the ripples that travel outward as a consequence. Shake a charged particle, and it will create waves of light. Disturb a massive object, and it will emit gravitational waves. We pick up light waves with our eyeballs, but gravitational waves are another matter. It took decades of effort and the construction of the colossal, miles-long detectors that make up the Laser Interferometer Gravitational-Wave Observatory (LIGO) to first sense a rumble in space-time in 2015 — one sent out by a collision between distant black holes.
Detecting a single graviton would be harder still, akin to noticing the effect of just one molecule in an ocean wave. How hard would it be? In a lecture in 2012, the eminent physicist Freeman Dyson considered gravitational waves from the sun, where the violent churning of matter inside the star should constantly send out mild tremors in space-time. Occasionally, one of the gravitons in these ripples would strike an atom in a detector and kick an electron into a higher energy level. Dyson calculated that in a detector as large as Earth, running for the 5-billion-year lifetime of the sun, such an effect might be seen just four times.
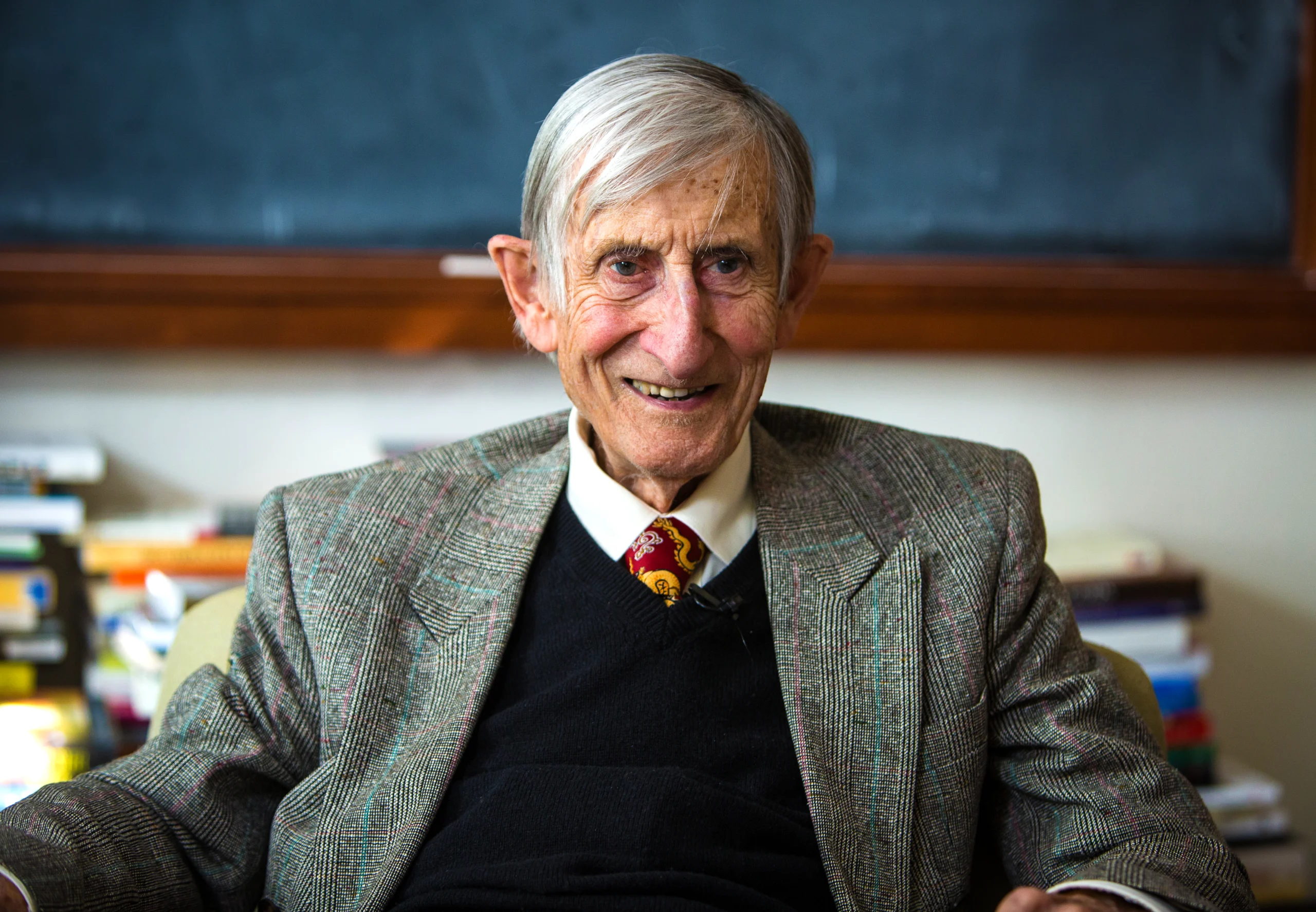
A calculation by the late physicist Freeman Dyson, pictured in his office at the Institute for Advanced Study, suggested that individual gravitons will never be detected.
D. Komoda
In the dozen years since Dyson’s remarks, two experimental developments have made the situation less dire. First, LIGO began detecting gravitational waves regularly from black hole collisions, and occasionally from colliding neutron stars. These events shake space-time far more intensely than the sun’s internal agitation does — providing a deluge of gravitons as opposed to Dyson’s trickle. And second, experimentalists have grown more capable of eliciting and measuring quantum phenomena.
Igor Pikovski, a theoretical physicist now at the Stevens Institute of Technology in New Jersey, has been mulling over these developments since 2016. At the time, he and three collaborators noted that a vat of superfluid helium — which displays quantum properties despite having a large mass — could be set up to reverberate in response to certain gravitational waves.
It would take another conceptual leap to go from a gravitational wave detector to a detector for individual gravitons. In the recent paper, which appeared in Nature Communications in August, Pikovski and his co-authors outlined how the graviton detector would work.
First, take a 15-kilogram bar of beryllium (or some similar material) and cool it almost all the way to absolute zero, the minimum possible temperature. Sapped of all heat, the bar will sit in its minimum-energy “ground” state. All the atoms of the bar will act together as one quantum system, akin to one hulking atom.
Then, wait until a gravitational wave from deep space passes by. The odds that any particular graviton will interact with the beryllium bar are low, but the wave will contain so many gravitons that the overall odds of at least one interaction are high. The group calculated that approximately one in three gravitational waves of the right sort (neutron star collisions work best since their mergers last longer than black hole mergers) would make the bar ring with one quantum unit of energy. If your bar reverberates in concert with a gravitational wave confirmed by LIGO, you will have witnessed a quantized event caused by gravity.
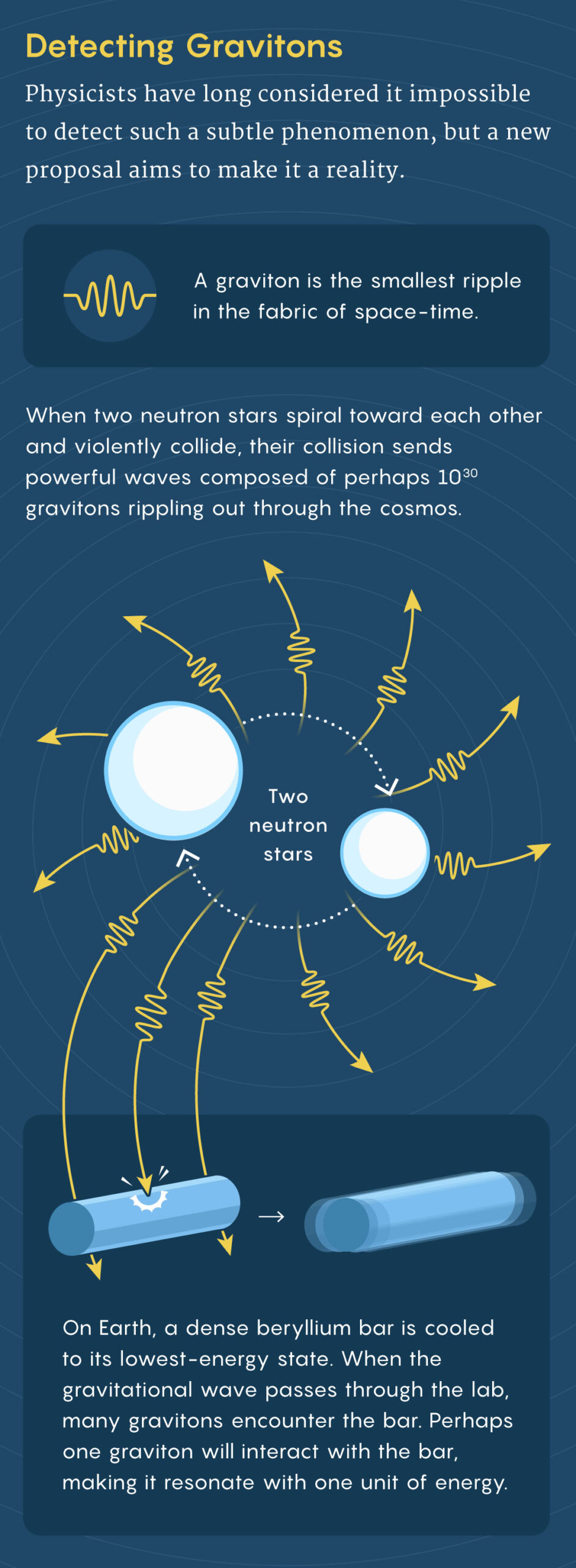
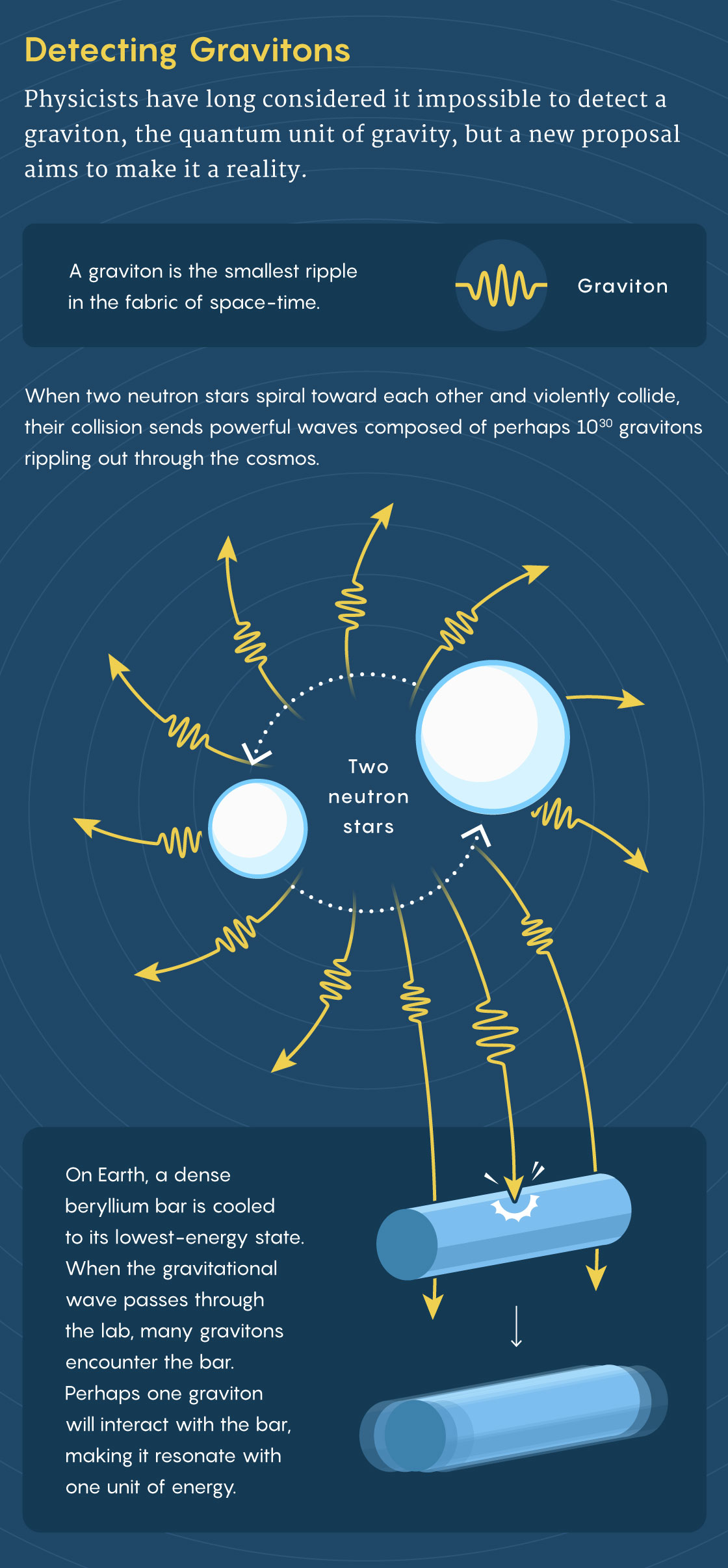
Mark Belan/ Quanta Magazine
“It would be our first window into where quantum gravity matters,” Pikovski said.
Among a handful of engineering hurdles involved in opening that window, the highest would be putting a heavy object into its ground state and sensing it jumping to its next-lowest-energy state. One of the groups pushing the state of the art on this front is at ETH Zurich, where Fadel and his collaborators cool tiny sapphire crystals until they display quantum properties. In 2023, the team succeeded in putting a crystal into two states simultaneously — another hallmark of a quantum system. Its mass was 16 millionths of a gram — heavy for a quantum object, but still half a billion times lighter than Pikovski’s bar. Nevertheless, Fadel considers the proposal to be achievable. “It wouldn’t be too crazy,” he said.
Pikovski’s experiment — like Dyson’s — emulates the very experiment that prompted Einstein to propose in 1905 that light is quantized, a watershed moment in the history of quantum mechanics. “If carried through, it would bring the state of the art in the case for gravitons to the same level that it was for photons in 1905,” Wilczek said.
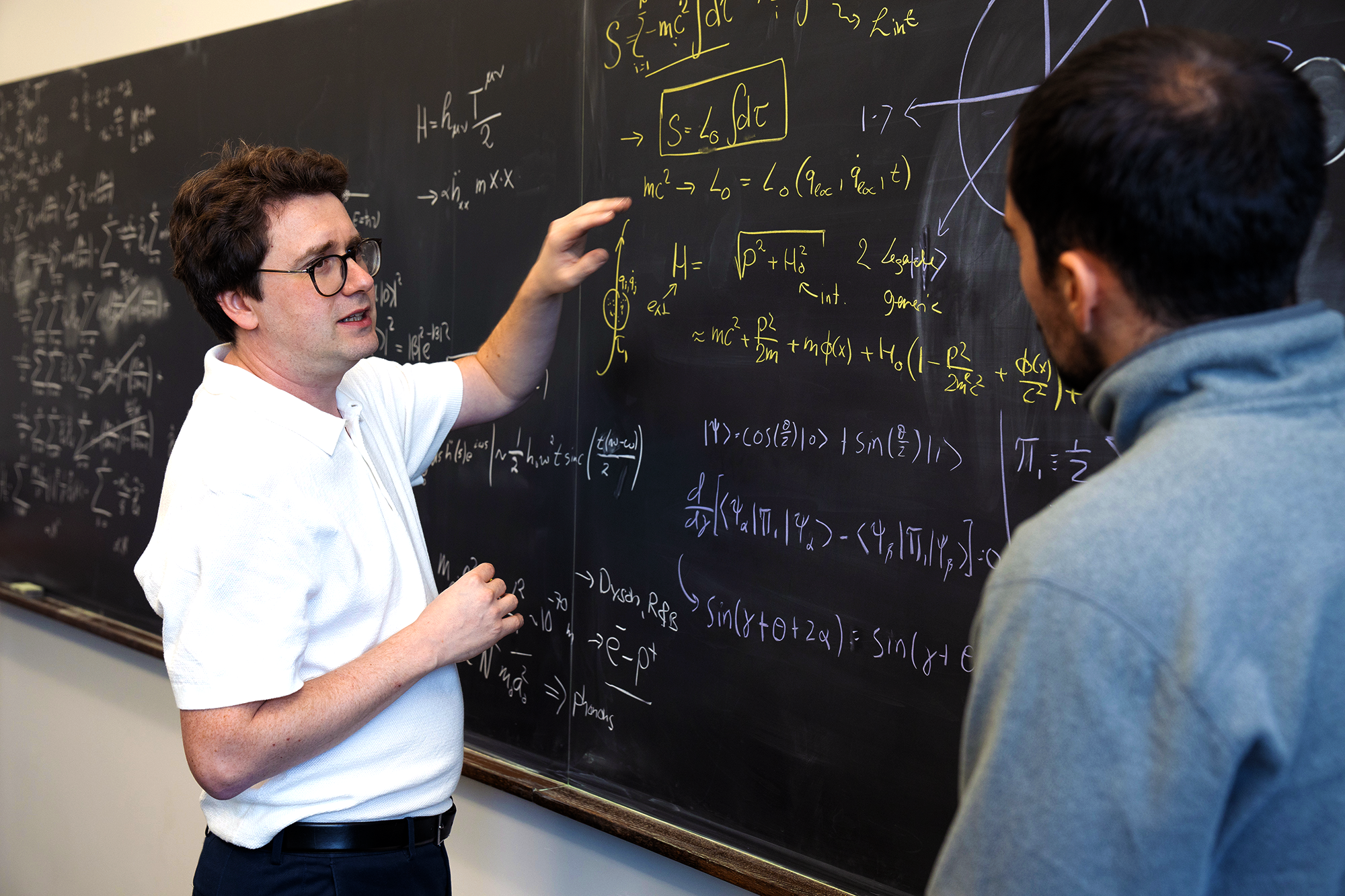
Igor Pikovski, a physicist at the Stevens Institute of Technology, has proposed a way to detect a quantized response to a gravitational wave.
Ian Reitz
Textbooks often credit Einstein’s paper with establishing the photon’s existence. But the real story is far more interesting. At the time, many physicists rejected Einstein’s theory. Some wouldn’t come around for two decades. In their view, the experiment fell far short of conclusive proof. It was, rather, an opening argument in a decades-long war fought to determine the true nature of light.
The Real Story of the Photon
Physicists saw the first cracks opening up in their classical understanding of reality in the closing years of the 19th century. J.J. Thomson discovered that electric currents come in discrete chunks of charge called electrons. Meanwhile, physicists were puzzling over a string of experiments by Heinrich Hertz and others that used light to make a current flow — a phenomenon that came to be called the photoelectric effect.
The puzzle was that when they directed dim beams of light at a metal plate, sometimes an electric current flowed across the plate and sometimes it didn’t. In the pre-quantum world this was hard to explain. It was believed that any wave should create at least a small current, and brighter waves should create larger currents. Instead, physicists found that there was a special color of light — a frequency — that got a current to flow. Only waves of that frequency or higher could start a current. Brightness had little to do with it.
Einstein proposed a solution in 1905: A wave of light is made of many discrete units called “quanta,” each with energy related to the wave’s frequency. The higher the frequency of the wave, the more energetic its quanta. And the brighter the wave, the more quanta there are. If you try to start an electric current in a metal plate with low-frequency red light, you’ll be no more successful than if you tried to knock over a refrigerator with ping-pong balls; no number would suffice. But using higher-frequency blue light is like switching to boulders. Each of those units has enough oomph to excite an electron, even in dim light with very few of them.
Einstein’s theory was met with skepticism. Physicists felt fiercely protective of James Clerk Maxwell’s then-40-year-old theory of light as an electromagnetic wave. They had seen light refracting, diffracting, and doing all the things waves do. How could it be made up of particles?
Even after Einstein won the 1921 Nobel Prize in Physics for his theory of the photoelectric effect, debate continued among physicists. The effect suggested that something is quantized; otherwise there wouldn’t be a minimum threshold required to get electrons flowing. But some physicists, including Niels Bohr, who is considered one of the founders of quantum theory, continued to explore the possibility that only the matter was quantized, not the light. Today, this type of theory is called “semiclassical” because it describes a classical field interacting with quantized matter.
To see how a semiclassical theory can explain the photoelectric effect, imagine a kid on a swing. They’re kind of like an electron in a metal. They have a ground state (not swinging) and an excited state (swinging). A classical wave is like giving the kid a series of pushes. If the pushes come at some random frequency, nothing happens. The kid might bounce around a little, but they will basically stay in their ground state. It’s only when you push with just the right frequency — the swing’s “resonant” frequency — that the kid accumulates energy and starts swinging. (Electrons in a metal are a little different; they resonate with a whole continuous “band” of frequencies instead of just the one. But the upshot is the same: Any wave below that frequency band does nothing, whereas any wave in that frequency band excites electrons and makes a current flow.)
Einstein was eventually vindicated, but not on the strength of the photoelectric effect alone. Later experiments that collided electrons and photons like projectiles found that momentum, too, came in chunks. This research eventually ruled out the main alternative — a semiclassical theory of light and matter from Bohr and his collaborators. In 1925, seeing the data, Bohr agreed to “give our revolutionary efforts as honorable a funeral as possible” and welcomed light into the quantum fold. Light quanta became known as photons.
Few doubted the photon after 1925, but physicists are nothing if not thorough. Just because no one could think of a viable semiclassical theory didn’t mean one didn’t exist. The final proof that photons are real came in the late 1970s, when quantum optics researchers showed that light arrived at a detector in a pattern no semiclassical theory could mimic. The experiments were akin to firing a photon gun once per second and confirming that the detector clicked once per second in response. The photon wars ended with a whimper.
“There were just mountains of evidence that this photon concept was useful and vital,” Wilczek said.
The Graviton Wars Begin
In August of 2023, Daniel Carney and his collaborators fired the first shot in a new war.
It started when Carney’s colleague Nicholas Rodd had an insight similar to Pikovski’s, about a possible way to detect a graviton. “We got super pumped,” said Carney, a physicist at Lawrence Berkeley National Laboratory.
But when he and his collaborators dug into the literature, they uncovered the messy history of the photon, and the lengths to which quantum optics researchers had gone in the 1970s to close the final loopholes. They translated those more stringent tests into the gravitational context and found that Dyson had been right. Really proving quantumness by detecting lone gravitons one after another — as opposed to plucking one out of a tsunami, in the style of Pikovski’s proposal — would indeed take planetary-scale machinery.
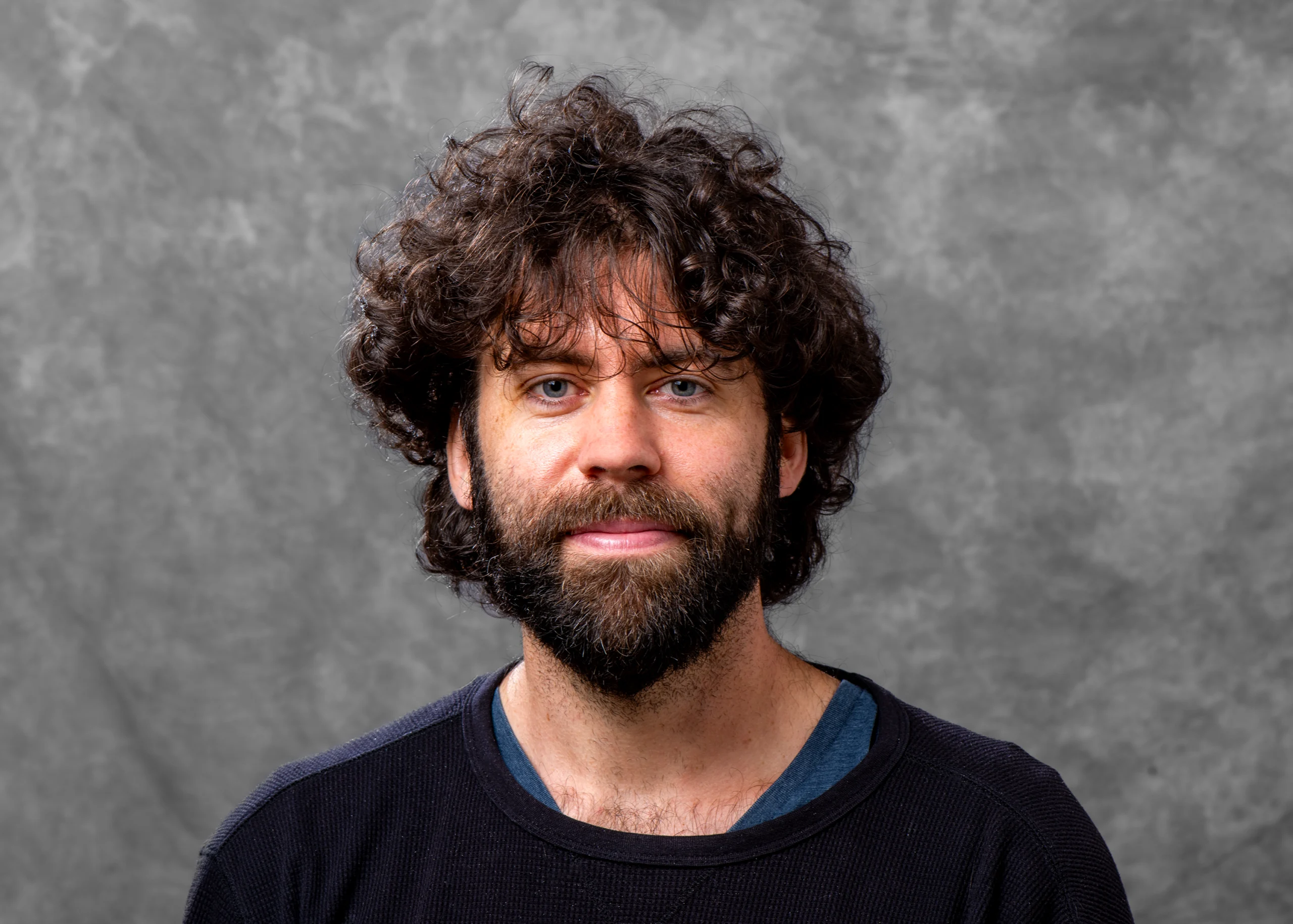
Daniel Carney, a physicist at Lawrence Berkeley National Laboratory, argues that a proposed experiment would not offer conclusive proof of quantum gravity.
Lawrence Berkeley National Laboratory
“It was crazy to have to revise your hypothesis by 100% really fast,” Carney said.
Now graviton chasers find themselves in a peculiar position. On the main facts, everyone is in agreement. One, detecting a quantum event sparked by a gravitational wave is — surprisingly — possible. And two, doing so would not explicitly prove that the gravitational wave is quantized. “Could you make a classical gravitational wave that would produce the same signal? The answer is yes,” said Carney, who along with two co-authors analyzed this type of experiment in Physical Review D in February.
How much physicists feel they would learn from the experiment varies. To some, it would strongly suggest that gravity is a quantum force because the alternative — a semiclassical theory of gravity and matter — is disfavored on other grounds. Such theories violate the conservation of energy, for instance. If the beryllium bar gains one quantum of energy, then energy conservation requires that the gravitational wave must have lost one quantum of energy — and therefore it must be quantized, too. (Einstein advanced this sort of argument for the photon in 1911.) Semiclassical theories save gravity’s classicality by sacrificing this revered principle.
“Unless you use very artificial interpretations,” Wilczek said, “it does tell you that you really should apply quantum mechanics to the gravitational wave.”
“If I want to see signatures of quantumness, it’s not my first goal to rule out these pathological things,” Pikovski said.
To physicists such as Carney, however, a mere strong suggestion that gravity is quantized isn’t all that informative. We already have an abundance of strong suggestions that all of reality is quantized, he says. What’s needed is proof — such as experiments that would close the remaining loopholes, no matter how bizarre they might seem.
“We’re so biased to think that everything is quantum that you should really be doing a lawyerly thing,” he said.
A Starting Point
While Pikovski’s proposal is not a loophole-closing experiment, many physicists would still like to see it happen. It would mark the dawn of an era of experimental quantum gravity, which until recently seemed quite far off.
“This is an exciting paper,” said Alex Sushkov, an experimental physicist at Boston University. “These are hard experiments, and we need bright, smart people to move in this direction.”
“We can take it as a starting point,” said Myungshik Kim, a physicist at Imperial College London.
It might motivate subsequent experiments that would take physicists deeper into the quantum gravity era, just as scattering experiments once took them deeper into the era of the photon. Physicists now know that quantum mechanics is much more than quantization. Quantum systems can take on combinations of states known as superpositions, for instance, and their parts can become “entangled” in such a way that measuring one reveals information about the other. Experiments establishing that gravity exhibits these phenomena would provide stronger evidence for quantum gravity, and researchers are already exploring what it would take to carry them out.
None of these tests of gravity’s quantum side are completely ironclad, but each would contribute some hard data regarding the finest features of the universe’s weakest force. Now a frigid quantum bar of beryllium appears to be a prime candidate for an experiment that will mark the first step down that long and winding road.