‘Last Hope’ Experiment Finds Evidence for Unknown Particles
Introduction
Twenty years after an apparent anomaly in the behavior of elementary particles raised hopes of a major physics breakthrough, a new measurement has solidified them: Physicists at Fermi National Accelerator Laboratory near Chicago announced today that muons — elementary particles similar to electrons — wobbled more than expected while whipping around a magnetized ring.
The widely anticipated new measurement confirms the decades-old result, which made headlines around the world. Both measurements of the muon’s wobbliness, or magnetic moment, significantly overshoot the theoretical prediction, as calculated last year by an international consortium of 132 theoretical physicists. The Fermilab researchers estimate that the difference has grown to a level quantified as “4.2 sigma,” well on the way to the stringent five-sigma level that physicists need to claim a discovery.
Taken at face value, the discrepancy strongly suggests that unknown particles of nature are giving muons an extra push. Such a discovery would at long last herald the breakdown of the 50-year-old Standard Model of particle physics — the set of equations describing the known elementary particles and interactions.
“Today is an extraordinary day, long awaited not only by us but by the whole international physics community,” Graziano Venanzoni, one of the leaders of the Fermilab Muon g-2 experiment and a physicist at the Italian National Institute for Nuclear Physics, told the press.
However, even as many particle physicists are likely to be celebrating — and racing to propose new ideas that could explain the discrepancy — a paper published today in the journal Nature casts the new muon measurement in a dramatically duller light.
The paper, which appeared just as the Fermilab team unveiled its new measurement, suggests that the muon’s measured wobbliness is exactly what the Standard Model predicts.
In the paper, a team of theorists known as BMW present a state-of-the-art supercomputer calculation of the most uncertain term that goes into the Standard Model prediction of the muon’s magnetic moment. BMW calculates this term to be considerably larger than the value adopted last year by the consortium, a group known as the Theory Initiative. BMW’s larger term leads to a larger overall predicted value of the muon’s magnetic moment, bringing the prediction in line with the measurements.
If the new calculation is correct, then physicists may have spent 20 years chasing a ghost. But the Theory Initiative’s prediction relied on a different calculational approach that has been honed over decades, and it could well be right. In that case, Fermilab’s new measurement constitutes the most exciting result in particle physics in years.
“This is a very sensitive and interesting situation,” said Zoltan Fodor, a theoretical particle physicist at Pennsylvania State University who is part of the BMW team.
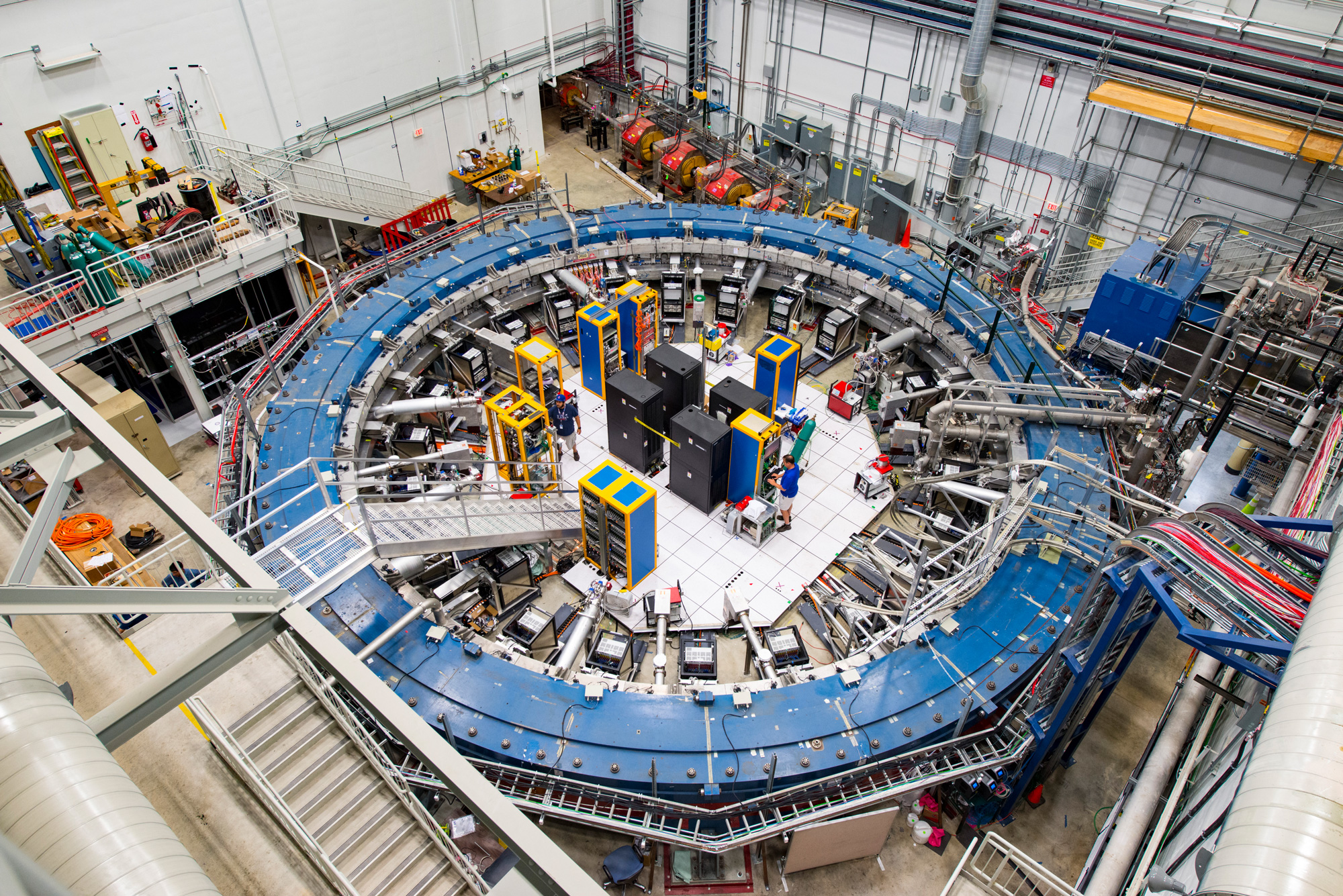
Electromagnets inside the 50-foot-wide Muon g-2 ring must be cooled to just a few degrees above absolute zero.
Reidar Hahn
BMW’s calculation itself is not breaking news; the paper first appeared as a preprint last year. Aida El-Khadra, a particle theorist at the University of Illinois who co-organized the Theory Initiative, explained that the BMW calculation should be taken seriously, but that it wasn’t factored into the Theory Initiative’s overall prediction because it still needed vetting. If other groups independently verify BMW’s calculation, the Theory Initiative will integrate it into its next assessment.
Dominik Stöckinger, a theorist at the Technical University of Dresden who participated in the Theory Initiative and is a member of the Fermilab Muon g-2 team, said the BMW result creates “an unclear status.” Physicists can’t say whether exotic new particles are pushing on muons until they agree about the effects of the 17 Standard Model particles they already know about.
Regardless, there’s plenty of reason for optimism: Researchers emphasize that even if BMW is right, the puzzling gulf between the two calculations could itself point to new physics. But for the moment, the past 20 years of conflict between theory and experiment appear to have been replaced by something even more unexpected: a battle of theory versus theory.
Momentous Muons
The reason physicists have eagerly awaited Fermilab’s new measurement is that the muon’s magnetic moment — essentially the strength of its intrinsic magnetism — encodes a huge amount of information about the universe.
A century ago, physicists assumed that the magnetic moments of elementary particles would follow the same formula as larger objects. Instead they found that electrons rotate in magnetic fields twice as much as expected. Their “gyromagnetic ratio,” or “g-factor” — the number relating their magnetic moment to their other properties — seemed to be 2, not 1, a surprise discovery later explained by the fact that electrons are “spin-1/2” particles, which return to the same state after making two full turns rather than one.
For years, both electrons and muons were thought to have g-factors of exactly 2. But then in 1947, Polykarp Kusch and Henry Foley measured the electron’s g-factor to be 2.00232. The theoretical physicist Julian Schwinger almost immediately explained the extra bits: He showed that the small corrections come from an electron’s tendency to momentarily emit and reabsorb a photon as it moves through space.
Many other fleeting quantum fluctuations happen as well. An electron or muon might emit and reabsorb two photons, or a photon that briefly becomes an electron and a positron, among countless other possibilities that the Standard Model allows. These temporary manifestations travel around with an electron or muon like an entourage, and all of them contribute to its magnetic properties. “The particle you thought was a bare muon is actually a muon plus a cloud of other things that appear spontaneously,” said Chris Polly, another leader of the Fermilab Muon g-2 experiment. “They change the magnetic moment.”
Samuel Velasco/Quanta Magazine
The rarer a quantum fluctuation, the less it contributes to the electron or muon’s g-factor. “As you go further into the decimal places you can see where suddenly the quarks start to appear for the first time,” said Polly. Further along are particles called W and Z bosons, and so on. Because muons are 207 times heavier than electrons, they’re about 2072 (or 43,000) times more likely to conjure up heavy particles in their entourage; these particles therefore alter the muon’s g-factor far more than an electron’s. “So if you’re looking for particles that could explain the missing mass of the universe — dark matter — or you’re looking for particles of a theory called supersymmetry,” Polly said, “that’s where the muon has a unique role.”
For decades, theorists have strived to calculate contributions to the muon’s g-factor coming from increasingly unlikely iterations of known particles from the Standard Model, while experimentalists measured the g-factor with ever-increasing precision. If the measurement were to outstrip the expectation, this would betray the presence of strangers in the muon’s entourage: fleeting appearances of particles beyond the Standard Model.
Muon magnetic moment measurements began at Columbia University in the 1950s and were picked up a decade later at CERN, Europe’s particle physics laboratory. There, researchers pioneered the measurement technique still used at Fermilab today.
High-speed muons are shot into a magnetized ring. As a muon whips around the ring, passing through its powerful magnetic field, the particle’s spin axis (which can be pictured as a little arrow) gradually rotates. Millionths of a second later, typically after speeding around the ring a few hundred times, the muon decays, producing an electron that flies into one of the surrounding detectors. The varying energies of electrons emanating from the ring at different times reveal how quickly the muon spins are rotating.
Samuel Velasco/Quanta Magazine
In the 1990s, a team at Brookhaven National Laboratory on Long Island built a 50-foot-wide ring to fling muons around and began collecting data. In 2001, the researchers announced their first results, reporting 2.0023318404 for the muon’s g-factor, with some uncertainty in the final two digits. Meanwhile, the most comprehensive Standard Model prediction at the time gave the significantly lower value of 2.0023318319.
It instantly became the world’s most famous eighth-decimal-place discrepancy.
“Hundreds of newspapers covered it,” said Polly, who was a graduate student with the experiment at the time.
Brookhaven’s measurement overshot the prediction by nearly three times its supposed margin of error, known as a three-sigma deviation. A three-sigma gap is significant, unlikely to be caused by random noise or an unlucky accumulation of small errors. It strongly suggested that something was missing from the theoretical calculation, something like a dark matter particle or an extra force-carrying boson.
But unlikely sequences of events sometimes happen, so physicists require a five-sigma deviation between a prediction and a measurement to definitively claim a discovery.
Trouble With Hadrons
A year after Brookhaven’s headline-making measurement, theorists spotted a mistake in the prediction. A formula representing one group of the tens of thousands of quantum fluctuations that muons can engage in contained a rogue minus sign; fixing it in the calculation reduced the difference between theory and experiment to just two sigma. That’s nothing to get excited about.
But as the Brookhaven team accrued 10 times more data, their measurement of the muon’s g-factor stayed the same while the error bars around the measurement shrank. The discrepancy with theory grew back to three sigma by the time of the experiment’s final report in 2006. And it continued to grow, as theorists kept honing the Standard Model prediction for the g-factor without seeing the value drift upward toward the measurement.
The Brookhaven anomaly loomed ever larger in physicists’ psyches as other searches for new particles failed. Throughout the 2010s, the $20 billion Large Hadron Collider in Europe slammed protons together in hopes of conjuring up dozens of new particles that might complete the pattern of nature’s building blocks. But the collider found only the Higgs boson — the last missing piece of the Standard Model. Meanwhile, a slew of experimental searches for dark matter found nothing. Hopes for new physics increasingly rode on wobbly muons. “I don’t know if it is the last great hope for new physics, but it certainly is a major one,” Matthew Buckley, a particle physicist at Rutgers University, told me.
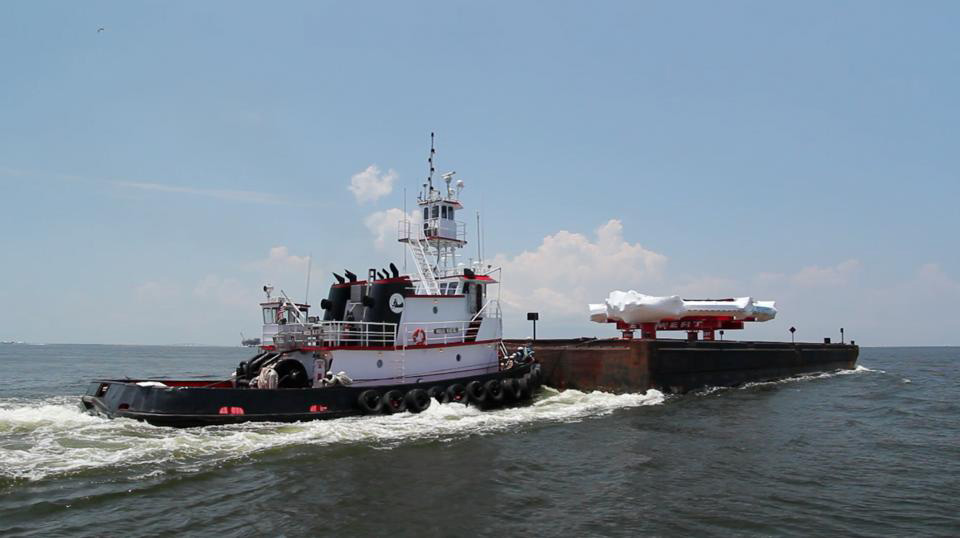
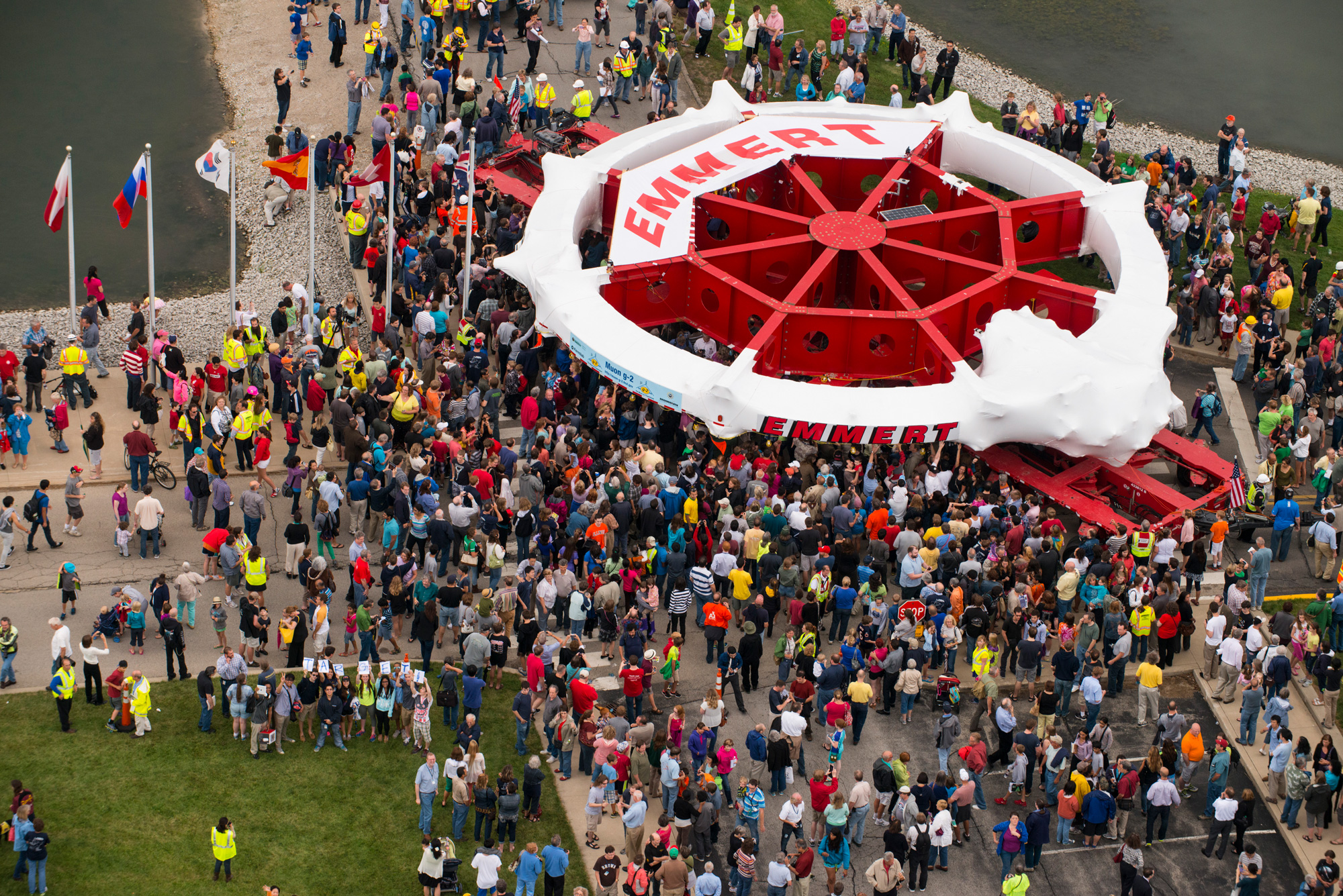
The original Muon g-2 experiment was constructed at Brookhaven National Laboratory on Long Island in the 1990s. Rather than build a new experiment from scratch, physicists used a series of barges and trucks to move the 700-ton electromagnetic ring down the Atlantic coast, across the Gulf of Mexico, and up the Mississippi, Illinois and Des Plaines rivers to the Fermi National Laboratory in Illinois. Thousands of people came out to celebrate its arrival in July 2013.
Darin Clifton/Ceres Barge; Reidar Hahn
Everyone knew that in order to cross the threshold of discovery, they would need to measure the muon’s gyromagnetic ratio again, and more precisely. So plans for a follow-up experiment got underway. In 2013, the giant magnet used at Brookhaven was loaded onto a barge off Long Island and shipped down the Atlantic Coast and up the Mississippi and Illinois rivers to Fermilab, where the lab’s powerful muon beam would let data accrue much faster than before. That and other improvements would allow the Fermilab team to measure the muon’s g-factor four times more accurately than Brookhaven had.
In 2016, El-Khadra and others started organizing the Theory Initiative, seeking to iron out any disagreements and arrive at a consensus Standard Model prediction of the g-factor before the Fermilab data rolled in. “For the impact of such an exquisite experimental measurement to be maximized, theory needs to get its act together, basically,” she said, explaining the reasoning at the time. The theorists compared and combined calculations of different quantum bits and pieces that contribute to the muon’s g-factor and arrived at an overall prediction last summer of 2.0023318362. That fell a hearty 3.7 sigma below Brookhaven’s final measurement of 2.0023318416.
But the Theory Initiative’s report was not the final word.
Uncertainty about what the Standard Model predicts for the muon’s magnetic moment stems entirely from the presence in its entourage of “hadrons”: particles made of quarks. Quarks feel the strong force (one of the three forces of the Standard Model), which is so strong it’s as if quarks are swimming in glue, and that glue is endlessly dense with other particles. The equation describing the strong force (and thus, ultimately, the behavior of hadrons) can’t be exactly solved.
That makes it hard to gauge how often hadrons pop up in the muon’s midst. The dominant scenario is the following: The muon, as it travels along, momentarily emits a photon, which morphs into a hadron and an antihadron; the hadron-antihadron pair quickly annihilate back into a photon, which the muon then reabsorbs. This process, called hadronic vacuum polarization, contributes a small correction to the muon’s gyromagnetic ratio starting in the seventh decimal place. Calculating this correction involves solving a complicated mathematical sum for each hadron-antihadron pair that can arise.
Uncertainty about this hadronic vacuum polarization term is the primary source of overall uncertainty about the g-factor. A small increase in this term can completely erase the difference between theory and experiment. Physicists have two ways to calculate it.
With the first method, researchers don’t even try to calculate the hadrons’ behavior. Instead, they simply translate data from other particle collision experiments into an expectation for the hadronic vacuum polarization term. “The data-driven approach has been refined and optimized over decades, and several competing groups using different details in their approaches have confirmed each other,” said Stöckinger. The Theory Initiative used this data-driven approach.
But in recent years, a purely computational method has been steadily improving. In this approach, researchers use supercomputers to solve the equations of the strong force at discrete points on a lattice instead of everywhere in space, turning the infinitely detailed problem into a finite one. This way of coarse-graining the quark quagmire to predict the behavior of hadrons “is similar to a weather forecast or meteorology,” Fodor explained. The calculation can be made ultra-precise by putting lattice points very close together, but this also pushes computers to their limits.
The 14-person BMW team — named after Budapest, Marseille and Wuppertal, the three European cities where most team members were originally based — used this approach. They made four chief innovations. First they reduced random noise. They also devised a way of very precisely determining scale in their lattice. At the same time, they more than doubled their lattice’s size compared to earlier efforts, so that they could study hadrons’ behavior near the center of the lattice without worrying about edge effects. Finally, they included in the calculation a family of complicating details that are often neglected, like mass differences between types of quarks. “All four [changes] needed a lot of computing power,” said Fodor.
The researchers then commandeered supercomputers in Jülich, Munich, Stuttgart, Orsay, Rome, Wuppertal and Budapest and put them to work on a new and better calculation. After several hundred million core hours of crunching, the supercomputers spat out a value for the hadronic vacuum polarization term. Their total, when combined with all other quantum contributions to the muon’s g-factor, yielded 2.00233183908. This is “in fairly good agreement” with the Brookhaven experiment, Fodor said. “We cross-checked it a million times because we were very much surprised.” In February 2020, they posted their work on the arxiv.org preprint server.
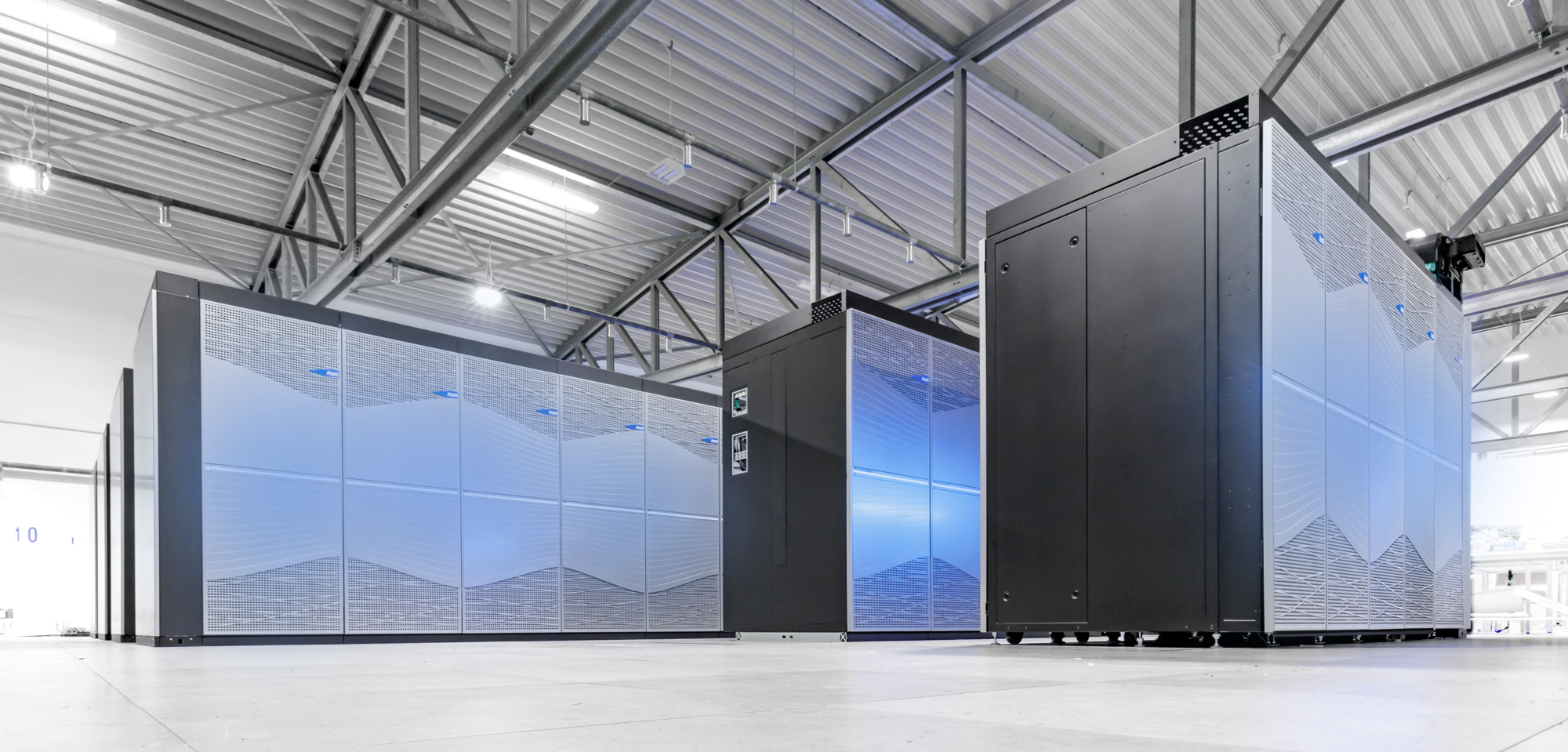
The JUWELS supercomputer at the Jülich Research Center in Germany is Europe’s most powerful. It was one of seven supercomputers used to calculate the anomalous magnetic moment of the muon.
Forschungszentrum Jülich / Ralf-Uwe Limbach
The Theory Initiative decided not to include BMW’s value in their official estimate for a few reasons. The data-driven approach has a slightly smaller error bar, and three different research groups independently calculated the same thing. In contrast, BMW’s lattice calculation was unpublished as of last summer. And although the result agrees well with earlier, less precise lattice calculations that also came out high, it hasn’t been independently replicated by another group to the same precision.
The Theory Initiative’s decision meant that the official theoretical value of the muon’s magnetic moment had a 3.7-sigma difference with Brookhaven’s experimental measurement. It set the stage for what has become the most anticipated reveal in particle physics since the Higgs boson in 2012.
The Revelations
A month ago, the Fermilab Muon g-2 team announced that they would present their first results today. Particle physicists were ecstatic. Laura Baudis, a physicist at the University of Zurich, said she was “counting the days until April 7,” after anticipating the result for 20 years. “If the Brookhaven results are confirmed by the new experiment at Fermilab,” she said, “this would be an enormous achievement.”
And if not — if the anomaly were to disappear — some in the particle physics community feared nothing less than “the end of particle physics,” said Stöckinger. The Fermilab g-2 experiment is “our last hope of an experiment which really proves the existence of physics beyond the Standard Model,” he said. If it failed to do so, many researchers might feel that “we now give up and we have to do something else instead of researching physics beyond the Standard Model.” He added, “Honestly speaking, it might be my own reaction.”
The 200-person Fermilab team revealed the result to themselves only six weeks ago in an unveiling ceremony over Zoom. Tammy Walton, a scientist on the team, rushed home to catch the show after working the night shift on the experiment, which is currently in its fourth run. (The new analysis covers data from the first run, which makes up 6% of what the experiment will eventually accrue.) When the all-important number appeared on the screen, plotted along with the Theory Initiative’s prediction and the Brookhaven measurement, Walton was thrilled to see it land higher than the former and pretty much smack dab on top of the latter. “People are going to be crazy excited,” she said.
Papers proposing various ideas for new physics are expected to flood the arxiv in the coming days. Yet beyond that, the future is unclear. What was once an illuminating breach between theory and experiment has been clouded by a far foggier clash of calculations.
It’s possible that the supercomputer calculation will turn out to be wrong — that BMW overlooked some source of error. “We need to have a close look at the calculation,” El-Khadra said, stressing that it’s too early to draw firm conclusions. “It is pushing on the methods to get that precision, and we need to understand if the way they pushed on the methods broke them.”
That would be good news for fans of new physics.
Interestingly, though, even if the data-driven method is the approach with an unidentified problem under the hood, theorists have a hard time understanding what the problem could be other than unaccounted-for new physics. “The need for new physics would only shift elsewhere,” said Martin Hoferichter of the University of Bern, a leading member of the Theory Initiative.
Researchers who have been exploring possible problems with the data-driven method over the past year say the data itself is unlikely to be wrong. It comes from decades of ultraprecise measurements of 35 hadronic processes. But “it could be that the data, or the way it is interpreted, is misleading,” said Andreas Crivellin of CERN and other institutions, a coauthor (along with Hoferichter) of one paper studying this possibility.
It’s possible, he explained, that destructive interference happens to reduce the likelihood of the hadronic processes arising in certain electron-positron collisions, without affecting hadronic vacuum polarization near muons; then the data-driven extrapolation from one to the other doesn’t quite work. In that case, though, another Standard Model calculation that’s sensitive to the same hadronic processes gets thrown off, creating a different tension between the theory and data. And this tension would itself suggest new physics.
It’s tricky to resolve this other tension while keeping the new physics “elusive enough to not have been observed elsewhere,” as El-Khadra put it, yet it’s possible — for instance, by introducing the effects of hypothetical particles called vector-like leptons.
Thus the mystery swirling around muons might lead the way past the Standard Model to a more complete account of the universe after all. However things turn out, it’s safe to say that today’s news — both the result from Fermilab, as well as the publication of the BMW calculation in Nature — is not the end for particle physics.
This article was reprinted on Wired.com.