Major Quantum Computing Strategy Suffers Serious Setbacks
Introduction
In 2018, researchers at the forefront of an entirely new approach to building quantum computers published, in the journal Nature, what looked to be a landmark achievement. Existing quantum computers are notoriously fragile, their quantum bits — qubits — prone to incurring random errors. But if the qubits could be made from strange configurations of electrons with the exotic name of Majorana zero-mode (MZM) quasiparticles, errors simply couldn’t occur. An MZM qubit can no more suffer a random error than you can separate the links of a chain without cutting them — the basic principles of topology, the mathematics of shape, protects against it.
These “topological” qubits are extremely difficult to build, but despite the technical challenges, some researchers are convinced that they are the only path to building a useful quantum computer with many hundreds or thousands of qubits. Microsoft, for one, is staking its main quantum computing strategy on topological qubits.
That’s one reason why the 2018 Nature paper garnered so much attention. A team led by Leo Kouwenhoven, a physicist at the Delft University of Technology in the Netherlands, said that they had found the definitive signature of MZM quasiparticles in indium antimonide nanowires. Their paper was heralded, with much press fanfare, as the dawn of topological quantum computing. In 2019, Microsoft opened its own quantum laboratory on the Delft campus, with Kouwenhoven as its director.
Then things started to fall apart. Later that year, Sergey Frolov, a physicist at the University of Pittsburgh, and his collaborator Vincent Mourik of the University of New South Wales in Australia were doing similar work in their own labs. (Both Frolov and Mourik are former members of Kouwenhoven’s group.) Frolov and Mourik found they couldn’t reproduce the Delft results. That October the duo asked Kouwenhoven’s group for their raw data, and in December they found some odd inconsistencies: It looked as though some of the plots had been manipulated, and the paper’s claims weren’t borne out when the full range of measurements was taken into account.
Faced with these problems, Kouwenhoven’s group replotted their data and found the conclusions no longer stood up. In March 2021, at Kouwenhoven’s request, Nature retracted the paper. The group wrote in their retraction that they could “no longer claim the observation of a quantized Majorana conductance.” They added an apology “for insufficient scientific rigour in our original manuscript.”
The incident triggered an investigation by an independent committee. The investigation concluded that there was no evidence of fraudulent data fabrication or manipulation. The authors had simply fooled themselves by zooming in only on the results that showed them what they hoped to see. “The research program the authors set out on is particularly vulnerable to self-deception, and the authors did not guard against this,” the authors of the report wrote.
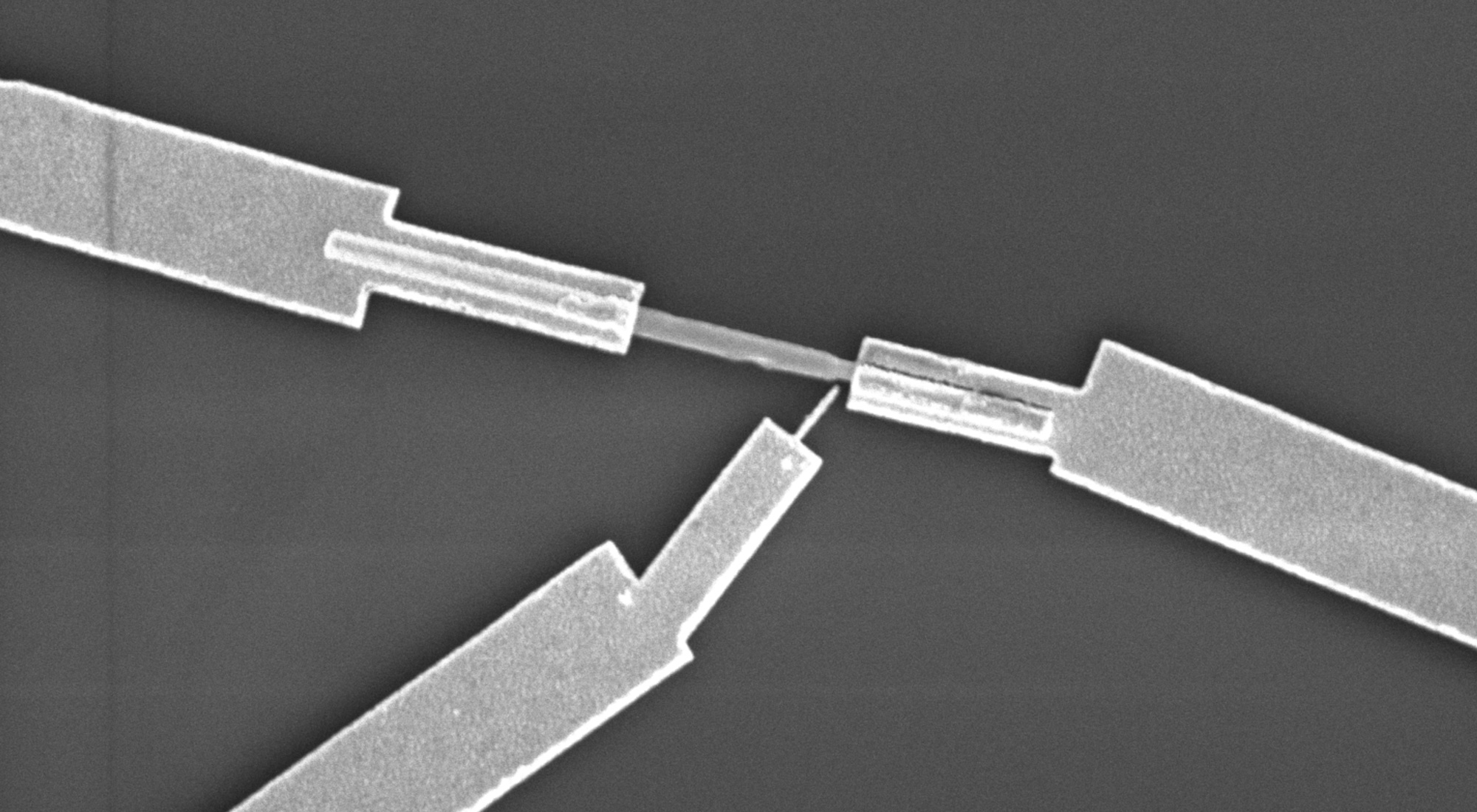
This scanning electron microcopy image reveals a nanowire used for Majorana experiments. The wire is approximately 1 micrometer long.
Marco Valentini
“It was an unfortunate incident of overzealousness combined with being careless,” said Patrick Lee, a physicist at the Massachusetts Institute of Technology and a member of the committee.
The retraction, along with other recent high-profile examples of related work that fell apart under closer inspection, has exposed an additional challenge at the heart of topological quantum computing research: Not only is it extremely difficult to build a topological qubit, but no one is sure how to even spot one. The quantum rules that lead to MZM quasiparticles also allow the creation of other weird quantum states — states that mimic Majorana particles but can’t be used as the basis for a quantum computer.
Because of these and other hurdles, the field of topological quantum computing has entered a period of self-reflection, if not outright crisis. “I’ve become concerned that, after a series of false starts, a significant fraction of the Majorana field is fooling itself,” Frolov wrote in a commentary in Nature in April. Yet despite this problem, even the field’s critics find the science too promising to ignore. “The physics behind the creation of Majoranas is well understood theoretically,” said Frolov during an online discussion. “Usually when that happens in condensed matter physics, the physical realization is not far behind. I am quite confident that within the next couple years, one or more groups will find strong evidence for them.”
The Power of Pairs
Majorana zero modes exemplify the way electrons in conducting materials can masquerade as different kinds of particles, with different charge, mass, mobility and collective behavior. They are “emergent effective particles made out of electrons,” said Charlie Marcus, a physicist at the Niels Bohr Institute and the director of the Microsoft quantum lab in Copenhagen. It’s rather like how we might describe economic activity as interactions of whole companies rather than of the people comprising them.
Perhaps the best-known example of an electron quasiparticle is the one responsible for superconductivity, where some metals and other materials at very low temperatures conduct electricity with zero resistance. In the simplest superconducting state, electrons seem to pair up as so-called Cooper pair quasiparticles. Even though they might be widely separated in space, the electrons in a Cooper pair act like a single particle as they move through the material. This pairing has a crucial consequence: Cooper pairs can all occupy the same lowest-energy quantum state, separated from the next state by an energy gap that the quasiparticles can’t jump across. As a result, Cooper pairs can’t easily be scattered into a different quantum state by collisions with atoms in the material’s crystal lattice, so they move without any resistance. You might say the quasiparticles are made of electrons that are protected from scattering.
MZMs are a different kind of electron quasiparticle. They are predicted to appear in a material, such as a semiconductor, that has an energy gap when electrons pair up in a different way. One of the simplest systems predicted to show this behavior, first proposed by the physicist Alexei Kitaev in the early 2000s, is a one-dimensional chain of electrons. At very low temperatures the electrons can pair up with their neighbors to become superconducting. But the electrons at each end of the chain have only half the partners. They become two halves of a single quasiparticle, widely separated in space. This quasiparticle can be thought of as existing right in the middle of the energy gap, at precisely zero energy — hence the “zero mode.”
The crucial point about zero-mode quasiparticles is that each one is made up of the electron states at both ends. So you can’t find out anything about it, or disturb it, by probing just one end. If the two end states could somehow be brought together in space, they would fuse to produce either an electron or nothing — a vacuum state. While they are separated, they are in a kind of quantum superposition of both states. So an MZM can act as a two-state bit for encoding quantum information: a qubit.
That the two halves of an MZM quasiparticle can fuse into nothing — an empty vacuum — accounts for their name. This self-annihilation is like that of a particle and antiparticle pair, except that here both members of the pair are identical. Hypothetical fundamental particles that are their own antiparticles were proposed as a possibility by the Italian physicist Ettore Majorana, working in Enrico Fermi’s group in Rome, in 1937. No such particle has ever been seen. MZMs are their quasiparticle equivalent, illustrating how behaviors of particles seen or suggested in high-energy physics are now turning up in materials governed by quantum laws.
The problem is, not only has no one ever made an MZM qubit, no one has convincingly shown the existence of even a single MZM quasiparticle.
The Escape Artist
The first reported sighting of Majoranas in nanowires came in 2012 from Kouwenhoven’s team at Delft. Looking back, it was an early sign of just how difficult it would be to establish unambiguous evidence of these elusive quasiparticles.
To look for MZMs, researchers generally apply a voltage to nanoscale wires made of semiconducting material connected to a superconductor. They carefully plot the conductance through the wire. If it contains MZMs, then as the voltage changes, the conductance should rise in discrete quantized steps and peak at precisely zero voltage — the energy corresponding to the zero mode.
Kouwenhoven’s 2012 paper reported that they discovered this “zero-bias peak,” but other researchers soon began to worry that the signal wasn’t unique to Majoranas. For example, Eduardo Lee, a physicist now at the Autonomous University of Madrid, and co-workers showed in 2013 that such a signal could be mimicked by what are known as Andreev bound states (ABSs) in the energy gap where MZMs are thought to sit. Then earlier this year, Marco Valentini of the Institute of Science and Technology in Klosterneuburg, Austria, and colleagues repeated experiments like those at Delft and showed that this system exhibits a zero-bias peak due to a particular kind of ABS called a Yu-Shiba-Rusinov state — a quasiparticle that also appears at zero energy but can’t be used to make a topological qubit.
Such false positives have dogged the field. In addition to Kouwenhoven’s retracted 2018 paper, skeptics point to other examples where claimed sightings of MZMs have not been replicated. In March 2020, Marcus and colleagues reported evidence in Science that the authors said was “consistent with the emergence of Majorana zero modes.” But then in late July 2021, the journal published an “editorial expression of concern” about whether the data used in the study was wholly representative. The Niels Bohr Institute has launched an independent investigation into the research. Marcus and his colleagues stand behind the original paper.
The trouble, according to critics like Frolov, is that Majorana particles aren’t necessary to produce the signals being seen. “Yet, affirmative papers kept coming out without even mentioning alternative explanations,” he wrote in his Nature critique. (Frolov declined multiple requests to be interviewed for this article.) He accuses researchers of cherry-picking their data, and calls for more openness about sharing it. He has identified five diagnostic signatures of Majoranas. Most of the papers claiming sightings since 2012 show just one or two of these, he said — but none shows them all.
Yet the real problem, Marcus asserts, is not premature claims but the difficulty of verification. Marcus wants to do the best experiments possible to see whether Majoranas exist, but “there won’t ever be a day on which you say: It’s proved!” he said. “That’s just not the way experimental physics works. There’s always going to be some smart guy out there who said, ‘I thought of something else! It doesn’t pass all the Majorana tests!’ That goes on forever.”
The question then becomes: How do you build a quantum computer out of something that you can’t say for sure exists?
Braided Information
The key to quantum computing is that, during the computation, you must avoid revealing what information your qubits encode: If you look at a bit and say that it holds a 1 or a 0, it becomes merely a classical bit. So you must shield your qubits from anything that could inadvertently reveal their value. (More strictly, decide their value — for in quantum mechanics this only happens when the value is measured.) You need to stop such information from leaking out into the environment.
That leakage corresponds to a process called quantum decoherence. The aim is to carry out quantum computing before decoherence can take place, since it will corrupt the qubits with random errors that will destroy the computation.
Current quantum computers typically suppress decoherence by isolating the qubits from their environment as well as possible. The trouble is, as the number of qubits multiplies, this isolation becomes extremely hard to maintain: Decoherence is bound to happen, and errors creep in. So for any of the existing quantum computers like those made by IBM, Google and others, scaling up to large systems “will clearly require huge amounts of active error correction,” said Scott Aaronson, a computer scientist and quantum theorist at the University of Texas, Austin. Current proposals for doing that involve wiring up huge numbers of “physical qubits” into a single “logical qubit” with the capacity for error correction. Some estimates suggest that hundreds or even thousands of physical qubits would be needed for each logical qubit — a dizzying technical challenge.
Kitaev realized that Majorana quasiparticles could be made resistant to errors — that is, to decoherence — if the two halves at the ends of an electron chain are jumbled up so that the information encoded in each pair of end states can be kept perfectly hidden and can’t inadvertently leak into the environment to cause decoherence. Because this jumbling weaves together the particle trajectories — their timeline “threads” — it is called braiding.
Here’s how braiding works. If you bring two Majorana quasiparticles together, they collapse to either an electron or a vacuum state. Once that happens, you know what they are — their information is revealed. But now suppose that many Majorana-mode pairs are shuffled around like couples in a square dance, constantly changing partners while maintaining an appropriate social distance. As long as the dance goes on, explains Marcus, “you can never focus on a single Majorana and ask it which state it is in — that is, whether it will fuse to an electron or to vacuum. Because it will say, ‘I don’t know, who am I dancing with?’” In effect, braiding ensures that the information in Majorana pairs is no longer localized on any of them but is made nonlocal. You can never reveal it by interrogating one of them.
“As long as they stay away from each other and change partners, the game is on,” said Marcus. “Kitaev’s genius was to say, if the information is stored nonlocally, then no local measurement reveals the information. That predicts that this thing should be a very good qubit” — because it is protected from incurring errors by the topological nature of the braiding.
To carry out a quantum computation with such MZM qubits, you shift the braided threads around in a specified way, and then bring pairs together to see if they fuse to form an electron or vacuum — that’s the readout. If that’s done in the right way, it performs the computation, free of any errors.
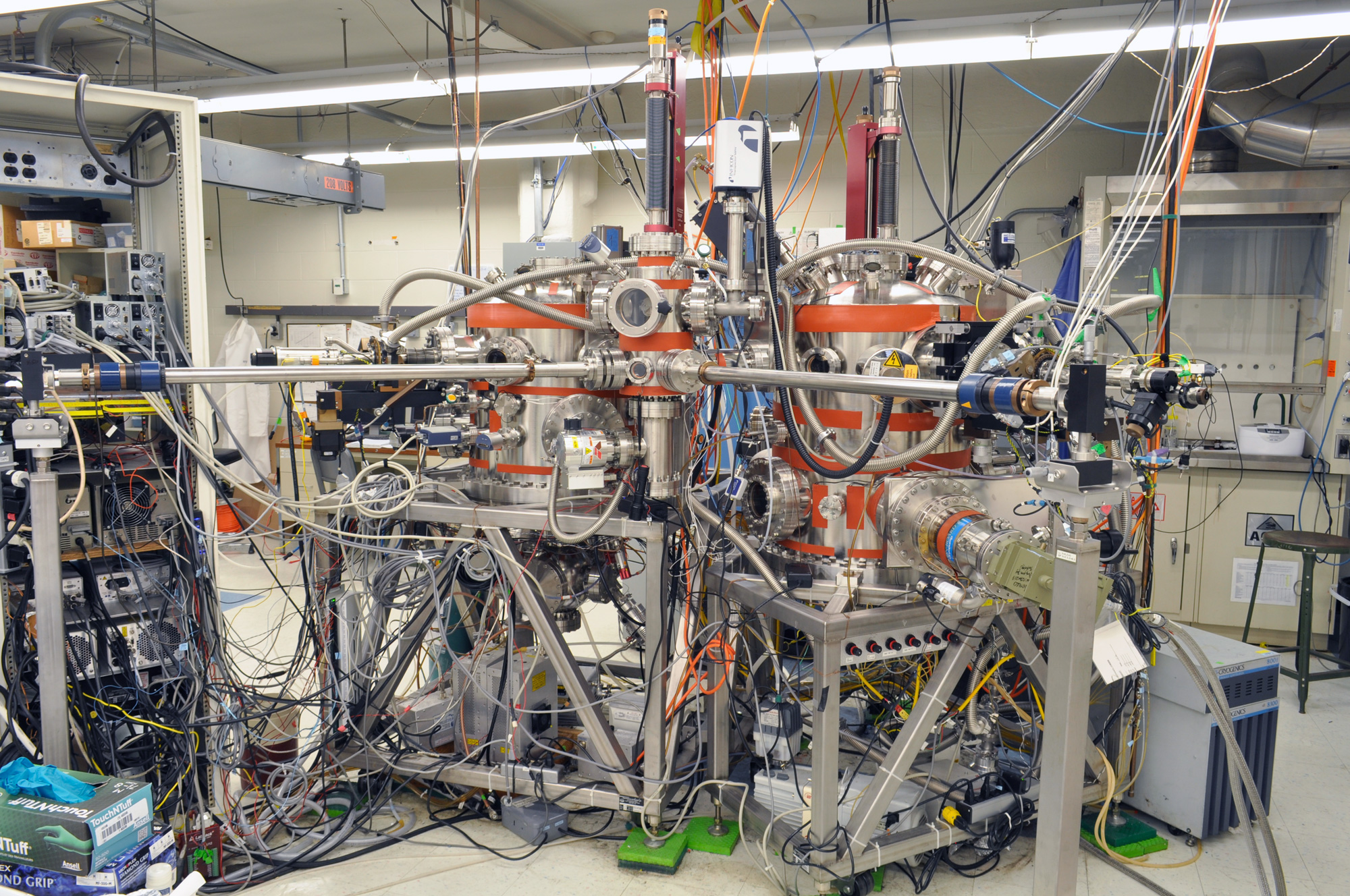
In order to create these extremely small devices, researchers developed this custom ultra-clean molecular beam epitaxy system.
Paul Rivenberg
Such error-resistant topological quantum computing using MZMs is “a challenging way to go, but not an impossible one,” said Lee. “Microsoft has gone out on a limb to make this gamble, [but] I think it is one worth taking.”
And if physicists are having an incredibly difficult time confirming the existence of MZM quasiparticles, perhaps they should simply forgo that step. In the end, Marcus thinks that experimentalists might need to stop trying to close all the loopholes and just go ahead and try braiding the system to see if a robust qubit emerges. After all, who cares if it’s definitively an MZM or some other quasiparticle, so long as it’s a good qubit?
Indeed, there are already proposals for using other quasiparticles as qubits too. For example, Christina Psaroudaki of the California Institute of Technology and Christos Panagopoulos of Nanyang Technological University in Singapore have suggested that skyrmions — vortex-like patterns in the orientation of spins in a magnetic material — might offer another way to encode and manipulate topologically protected quantum information.
Even though “the difficulties in finding MZMs, or more generally getting even the most basic building blocks of topological quantum computing to work in the lab, have clearly been a disappointment to many in the field,” said Aaronson, hope survives. He feels sure they’ll be found “sooner or later.” Valentini agrees, despite having shown that MZM signatures could be mistaken for other quasiparticles. “I strongly believe that MZMs will be created and detected,” he said.
But Aaronson adds that “whether this is a viable approach to quantum computing more broadly — and whether it will win over competing approaches — is still anyone’s guess.”
In early 2018, around the time that Nature published the doomed topological computing paper, Todd Holmdahl, the Microsoft vice president then in charge of their quantum computing efforts, predicted that they would have a working topological qubit by the end of the year, and a commercial quantum computer based on the technology in five years’ time. Although those predictions now seem extravagantly optimistic, they mirror the broader hype that has accompanied quantum computing over the past 10 years. More traditional approaches to quantum computers have enjoyed a degree of success, but they still face enormous challenges in scaling up. The best path to the long-promised quantum future remains unclear.
“It would be irresponsible of the physics community to decide now that we already know the only way,” said Ady Stern of the Weizmann Institute of Science in Rehovot, Israel. “We’re on page 10 of a thriller, and we’re trying to guess how it’s going to end.”
Correction: September 30, 2021
Some captions in the original version of this article misidentified the scientist who provided the photo as being the leader of the laboratory where the photo was taken. Nanowires were said to come from the lab of Marco Valentini, yet Valentini is a researcher in the laboratory of Georgios Katsaros. In addition, a device identified in Patrick Lee’s lab was actually in the lab of Jagadeesh S. Moodera, who partnered with Lee. Quanta regrets the errors.
Clarification: October 7, 2021
This article has been updated to clarify that Frolov declined to be interviewed for this article.