The Quantum Secret to Superconductivity
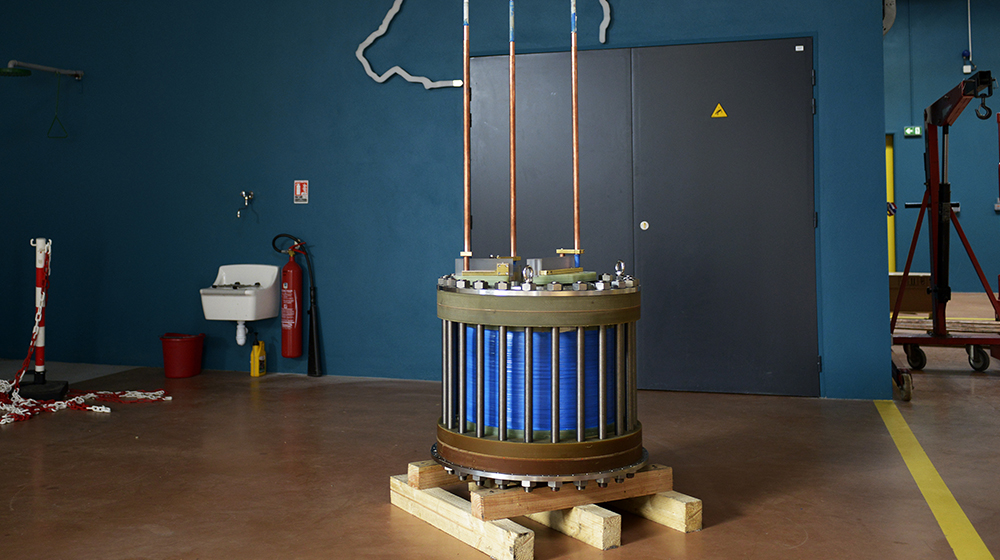
The energy equivalent of several kilograms of TNT surged into the coil, bathing the 0.003-carat crystal in its bore in one of the strongest magnetic fields ever generated.
From the magnet came a small boom like the sound of a foot stomping, said engineer Jérôme Béard — but thankfully, no explosion. His calculations held up.
With that magnetic blast and a subsequent series of identical ones executed last winter, researchers at the National Laboratory for Intense Magnetic Fields (LNCMI) in Toulouse, France, uncovered a key property of the crystal, a matte-black ceramic in a class of materials called cuprates that are the most potent superconductors known. The findings, reported today in the journal Nature, provide a major clue about the inner workings of cuprates, and may help scientists understand how these materials allow electricity to flow freely at relatively high temperatures.
“Technically amazing,” said J.C. Séamus Davis, an experimental physicist with appointments at Cornell University, St. Andrews University in Scotland, and Brookhaven National Laboratory who was not involved in the experiment. “The paper is a masterpiece.”
The experimental team, led by LNCMI staff scientist Cyril Proust and Louis Taillefer of the University of Sherbrooke in Canada, used their 90-tesla magnet — which creates a magnetic field nearly two million times as strong as the one enshrouding Earth — to momentarily strip away superconductivity in their cuprate sample. This revealed details of the underlying phase from which the behavior seems to arise.
With the veil lifted, the scientists discovered a sharp change in behavior at what appears to be a “quantum critical point” in cuprates, reminiscent of the freezing point of water. Theorists have long speculated that such a quantum critical point might exist, and that it could play a key role in superconductivity, said Andrey Chubukov, a condensed-matter theorist at the University of Minnesota. “One thing is to say this; another thing is to measure it,” Chubukov said.
Superconductivity is a phenomenon in which electricity flows without resistance from the material it moves through, so that no energy is lost in the process. It occurs when electrons (the negatively charged carriers of electricity) unite to form pairs, balancing each other’s properties in a way that allows them all to move in unison. The phase in which this happens is delicate, typically occurring only when a material is cooled to rock-bottom temperatures. But if wires could be engineered to act as superconductors at room temperature, experts say the lossless electrical transmission would greatly reduce global energy usage and usher in a host of new technologies, such as magnetically levitating vehicles and cheap water-purification systems.
The force driving superconductivity is strongest in cuprates. As IBM researchers Georg Bednorz and K. Alexander Müller discovered in 1986 (in work that earned them Nobel Prizes the following year), cuprates superconduct at much higher temperatures than other materials, suggesting that their electrons are paired by a different and stronger glue. But cuprates must still be cooled below minus 100 degrees Celsius before they become superconductive. The glue must be further strengthened if superconductors’ operating temperatures are to be dialed up. For 30 years scientists have asked: What is the glue — or, more precisely, the quantum mechanical interaction between electrons — that causes superconductivity to arise in cuprates?
While the detection of a quantum critical point does not definitively answer that question, “this has really clarified the situation,” said Subir Sachdev, a leading condensed-matter theorist at Harvard University. The finding knocks several proposals for the electron-pairing glue in cuprates out of the running. “There are now two prominent candidates for what’s happening,” Sachdev said.
One of the candidates, if verified, would enter the textbooks as a completely novel quantum phenomenon, with an exoticism that appeals to many theorists. But if the other, more conventional explanation of high-temperature superconductivity proves true, then, according to Davis, scientists will immediately know the key handle that needs to be turned to strengthen the effect. In that case, in the quest for room-temperature superconductivity, Davis said, “the route forward would be clear.”
Under the Dome
Proust, Taillefer and their collaborators set out eight years ago to blaze a trail to the center of the cuprate “phase diagram,” a map representing the hodgepodge of different phases exhibited by the materials as their properties are varied.
The two extreme ends of the map are well-understood: Pure, unadulterated cuprate crystals — mapped on the left side of the diagram — act as insulators, while cuprates that have been doped with many extra electrons or “holes” (deficits of electrons that behave like positively charged particles), mapped on the right, behave like metals. “The big fundamental question is,” Taillefer said, “How does the system go from insulator to metal?” Scientists become lost in the jumble of phases that occur at intermediate doping levels — including superconductivity, which rises like a dome in the middle of the phase diagram.
The map offers a clue: A line slopes up and to the left above the superconductivity dome, dividing two other, higher-temperature phases of the material. Extend this line downward to lower temperatures, and it strikes the base of the superconductivity dome exactly at its center point. Theorists have long suspected that the nature of this point might be the key to understanding superconductivity, which seems to form a bubble around it. Fifteen years ago, Taillefer and Proust, who was then a postdoctoral researcher in Taillefer’s lab, started to think about how to investigate this possible critical point. The problem was that the two phases they observed at higher temperatures, which seemed bound to meet at this point at a temperature of absolute zero, disappeared when superconductivity kicked in. In order to probe what happens during the transition from one phase to the other, the team had to find a way to stop the electrons in cuprates from forming superconducting pairs in the vicinity of the critical point.
To do this, the scientists needed a big magnet. Magnetic fields destroy superconductivity by exerting opposite forces on the electrons in each superconducting pair, breaking their connection. But the stronger the pairing glue in a superconductor, the harder it is to break. “With cuprates, the magnetic field you need to dissuade superconductivity is very high,” Proust said.
A Mighty Magnet
Magnets can only be as strong as the materials they are made of, which must withstand the enormous mechanical forces generated by tsunamis of electricity.
The 90-tesla magnet at LNCMI in Toulouse works by charging a bank of 600 capacitors, then discharging them all at once into a coil the size of a trash can. The coil is made of ultra-strong copper alloy reinforced with Zylon, a fiber stronger than Kevlar. For about 10 milliseconds, the flash flood of current generates a powerful magnetic field running through the coil’s bore. Although the LNCMI magnet can’t match the power of the 100-tesla magnet at Los Alamos National Laboratory in New Mexico, “we are able to make a very long pulse, two times longer than in Los Alamos,” enabling more precise measurements, Béard said.
As the engineers built the magnet, collaborators at the University of British Columbia prepared samples of a cuprate called yttrium barium copper oxide. They doped the samples with four different concentrations of holes, spanning from one side of the hypothesized critical point to the other. Upon cooling the samples to minus 223 degrees Celsius and blasting them with magnetic pulses, momentarily destroying superconductivity, they measured a property of the material that indicates the number of holes per atom that are involved in carrying electricity. Normally, this “carrier density” increases gradually as a function of doping. But at a critical point, it would be expected to change suddenly, indicating a spontaneous reorganization of the electrons in the crystal. And that’s what the scientists measured: a sharp, sixfold jump in the carrier density at 19 percent doping, the expected location of the critical point.
“There clearly is a hidden critical point right where Louis [Taillefer] says there is,” said Davis, who found indirect evidence for the existence of this point in 2014. “It points strongly to the idea that there is a sudden change to the electronic structure at that critical point.”
Quantum Critical Point
Unlike the freezing point of water, which is crossed by raising or lowering the water temperature, the critical point in cuprates is a “quantum critical point,” or a point of balance between two competing quantum mechanical states, at zero temperature. The quantum state that prevails to the left of the quantum critical point in the phase diagram causes the electrons to be “ordered,” or arranged into a pattern. The quantum effect that dominates on the right causes the electrons to be free-moving. But as the system approaches the critical point from either the left or the right, the amount of order in the system starts to fluctuate, due to competition between the two states. It is these fluctuations of order that are hypothesized to give rise to superconductivity in the vicinity of the quantum critical point. The question is: What kind of order is it?
For the past five years, researchers have suspected a type of order known as charge density waves — essentially, ripples of overly dense and under-dense regions of electrons. But the new experiment, as well as recent findings by Davis’ group, indicate that the charge density wave order dies out at a lower doping level, too far to the left of the quantum critical point. Now, two leading possibilities remain.
The more conventional option, proposed in the late 1980s by David Pines, Douglas Scalapino and other theorists, is antiferromagnetism, a type of order in which electrons alternate their spin directions in a checkerboard pattern — up, down, up, down, etc. Fluctuations in this checkerboard arrangement near the quantum critical point cause oppositely spinning electrons to become attracted to each other and pair up, giving rise to superconductivity. Several indirect observations support the antiferromagnetism hypothesis. According to Chubukov, because this order would be expected to set in at a quantum critical point, the new discovery is “the necessary missing link” in the antiferromagnetism explanation.
But if straightforward antiferromagnetism were the answer, physicists would have cracked the case decades ago. Experimenters have long tried and failed to detect antiferromagnetic order in the phase at the top left of the superconductivity dome — the presumed ordered phase to the left of the quantum critical point. “The trouble in the cuprates is, there is no long-range order that anyone can find,” said Stephen Julian, an experimental condensed-matter physicist at the University of Toronto. When experimenters look for the checkerboard pattern, they don’t see it.
However, defenders of the antiferromagnetic explanation point to the crystalline structure of cuprates, which are essentially stacked, two-dimensional sheets, and to a 1975 theorem known as the Mermin-Wagner theorem, which says that true, long-range antiferromagnetic order cannot develop in two-dimensional materials at non-zero temperatures. Instead, perhaps only patches of order develop, like sections of checkerboard, and these cannot be detected with existing experimental techniques. Long-range antiferromagnetic order only sets in at low temperatures, proponents say. The problem is that antiferromagnetism gets overridden by the phase it incites — superconductivity — and so still cannot be observed.
Not everyone thinks the Mermin-Wagner theorem is relevant. Davis points out that antiferromagnetic order has been detected in undoped cuprates, which have the same two-dimensional structure. The lack of antiferromagnetic order seen so far near the critical point has led some researchers to abandon this idea and support a more exotic theory put forward by Sachdev, which builds on concepts that Philip Anderson, a Nobel Prize winner and one of the founders of condensed-matter physics, advanced in the 1980s. Sachdev posits a kind of order in cuprates that isn’t seen in other materials. In this order, electrons form composites possessing fractions of spin and charge. Sachdev maintains that remnants of this order, which he has dubbed the fractionalized Fermi liquid or FL* state, form the precursor to high-temperature superconductivity.
Deciding whether the newfound quantum critical point is associated with antiferromagnetism or something more unusual like FL* will once again require powerful magnets. Experimenters are already working on ways to search for the checkerboard pattern of antiferromagnetic order at low temperatures, while using magnetic pulses to wipe out the superconductivity that arises there. “All these things will happen now,” Taillefer said. “It looks so much like an [antiferro]magnetic transition at that critical point, that that’s the question we need to answer.”
If antiferromagnetism turns out to be the electron-pairing glue in cuprates, then theorists will immediately focus on determining why the glue is so much stronger in these materials than in others, in hopes of further strengthening it. FL*, on the other hand, would provide theorists with a new set of dials altogether. Either way, many are optimistic that they are on track to raise the operating temperatures of superconductors. “I don’t think anybody believes there’s a fundamental limit” that prevents room-temperature superconductivity, Julian said. “The argument is how long it’s going to take us to get there. Some people think it’s just around the corner. Some people think it’s going to take a very long time.”
This article was reprinted on Wired.com.