Neuronal Scaffolding Plays Unexpected Role in Pain
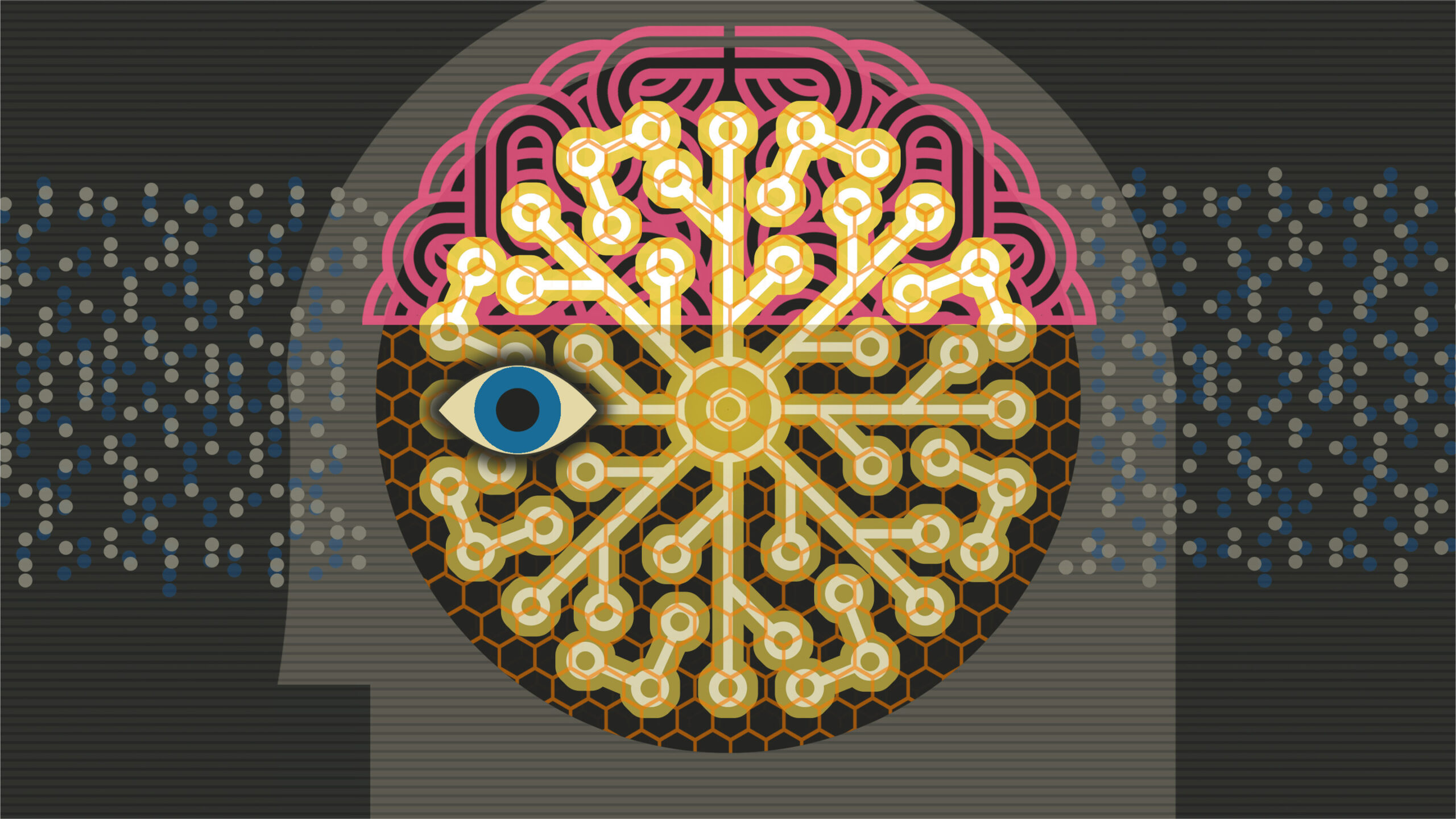
Gordon Studer for Quanta Magazine
Introduction
Neuroscientists, being interested in how brains work, naturally focus on neurons, the cells that can convey elements of sense and thought to each other via electrical impulses. But equally worthy of study is a substance that’s between them — a viscous coating on the outside of these neurons. Roughly equivalent to the cartilage in our noses and joints, the stuff clings like a fishing net to some of our neurons, inspiring the name perineuronal nets (PNNs). They’re composed of long chains of sugar molecules attached to a protein scaffolding, and they hold neurons in place, preventing them from sprouting and making new connections.
Given this ability, this little-known neural coating provides answers to some of the most puzzling questions about the brain: Why do young brains absorb new information so easily? Why are the fearful memories that accompany post-traumatic stress disorder (PTSD) so difficult to forget? Why is it so hard to stop drinking after becoming dependent on alcohol? And according to new research from the neuroscientist Arkady Khoutorsky and his colleagues at McGill University, we now know that PNNs also explain why pain can develop and persist so long after a nerve injury.
Neural plasticity is the ability of neural networks to change in response to experiences in life or to repair themselves after brain injury. Such opportunities for effortless change are known as critical periods when they occur early in life. Consider how easily babies pick up language, but how difficult it is to learn a foreign language as an adult. In a way, this is what we’d want: After the intricate neural networks that allow us to understand our native language are formed, it’s important for them to be locked down, so the networks remain relatively undisturbed for the rest of our lives.
This means that after a critical period, neural networks become resistant to change, and PNNs are a major reason why. They form over neurons and lock neural network wiring in place at the end of the critical period. This happens most often between the ages of 2 and 8, but PNNs also form on neurons in adulthood in association with behaviors that are hard to break, or in the formation of long-term memories. If we could delay the closure of critical periods, or somehow reopen them later in life, this would restore youthful neural plasticity, promote recovery from injury and undo difficult neurological disorders that are resistant to change.
Recent research shows that this can indeed be done, simply by manipulating PNNs. For example, keeping an animal in complete darkness slows the development of PNNs on vision neurons, keeping open the critical period for neural plasticity to correct vision problems much longer. Chemical agents and genetic manipulation can also degrade PNNs and reopen critical periods, and researchers have done this to make mice forget memories that caused them PTSD (in their case, memories of an electric shock administered right after they heard a tone).
It’s also possible to stimulate the growth of PNNs. This happens when someone drinks alcohol to excess, which results in the formation of these nets on neurons involved in addiction. The coating is believed to protect neurons from the chemical toxicity of the alcohol, but it also locks in the thought process that triggers an overpowering urge to drink.
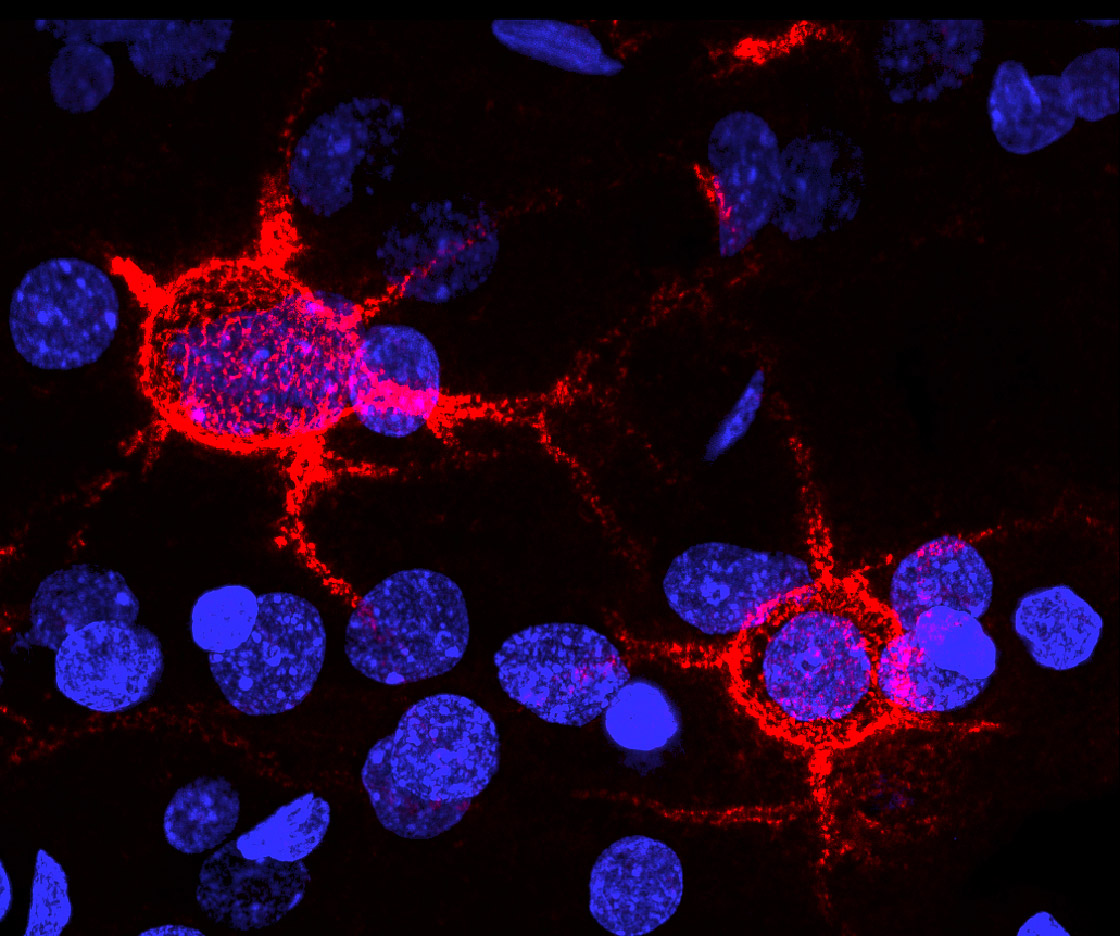
Perineuronal nets (in red) surround neurons in a mouse brain (with nuclei stained blue). New research shows similar nets in the spinal cord also help regulate how a brain perceives pain.
Kuznetsova Svetlana, Melnikova Anastasiya, Arnst Nikita
While neuroscientists have learned about these aspects of PNNs over the past few decades, the influence of PNNs on chronic pain was an unexpected recent discovery. This work, which further extends the nets’ influence beyond critical periods, not only improves our understanding of the basic science of pain, but also gives us a better picture of PNNs themselves.
Chronic pain, which persists long after an injury, reflects a change in neuronal circuitry that can be difficult to overcome. When something hurts, our whole body gets involved. Specialized pain neurons throughout the body transmit neural impulses into the spinal cord, where they are relayed to the brain. This means the spinal cord plays a major role in our sense of pain; indeed, doctors often manage the pain of childbirth by administering an epidural, which involves injecting anesthetics into the space surrounding the lumbar spinal cord, blocking neural impulses from reaching the brain.
Now imagine if instead of suppressing neural transmission at this point, a nerve injury made those neurons hypersensitive. Even a gentle touch in the affected area would cause a barrage of neuronal impulses to travel up the spinal cord, registering as intense pain. Previous research identified several mechanisms that can cause such hypersensitization, but no one expected PNNs to be involved.
A few years ago, however, Khoutorsky saw a paper reporting that PNNs were coating certain small neurons in a brain region where pain information is transmitted. These “inhibitory interneurons” form synapses on the pain neurons, suppressing their ability to transmit pain signals. Khoutorsky wondered if PNNs might be doing something similar at the critical pain relay point inside the spinal cord, and he asked his graduate student Shannon Tansley to look into it. “At that time nothing was known,” Khoutorsky said.
Tansley did indeed find that PNNs were encasing certain neurons in the spinal cord where it relays pain signals to the brain. The neurons have long axons (the “tail” that sends signals to the next cell in line) that point up the spinal cord to the brain. They also have a set of inhibitory interneurons attached to them through small holes in the PNN, and the inhibitory neurons can squelch the firing of the long projecting neurons, shrinking the signal reaching the brain and blunting the sensation of pain. Tansley discovered, to her surprise, that only these inhibitory neurons in the spinal cord relay point were coated with PNNs.
This finding inspired Khoutorsky’s team to undertake experiments on laboratory mice to determine if these nets were somehow involved in chronic pain after a peripheral nerve injury. They cut branches of a mouse’s hind leg nerve, known as the sciatic, while it was under general anesthesia. This mimics sciatic injuries in people, which are known to cause persistent pain. Days later, Khoutorsky’s team measured the mouse’s pain threshold with non-harmful tests, such as timing how quickly it recoiled from a warmed surface. As expected, the team saw the mouse display sharply increased pain sensitivity — but they also noticed that the PNNs around the projecting neurons had dissolved. Just as the brain’s changes during critical periods affects PNNs, the abrupt changes after nerve injury in the mouse had modified the PNNs in its spinal cord’s pain circuit.
The team then figured out what was causing the nets’ destruction: microglia, the brain and spinal cord cells that initiate repairs after disease and injury. To test the connection between microglia and pain, the team turned to mice with virtually no microglia (made possible with genetic engineering) and performed the same operation. In these mice, the PNNs remained intact after the sciatic nerve surgery and, remarkably, the mice did not become hypersensitive to painful stimuli. To confirm the connection, the team used various means to dissolve the nets, which raised the mice’s sensitivity to pain.
This proved that the PNNs were directly suppressing pain sensitivity. By measuring synaptic transmission with electrodes, Khoutorsky’s team even found out how it works. Degrading the PNNs caused a chain reaction that resulted in increased signaling from the projecting neurons that send pain signals to the brain: When the microglia responding to the nerve injury dissolved the PNNs, this weakened the influence of the inhibitory neurons that normally dampen the firing of the brain-projection neurons. Losing their inhibitory brakes meant runaway neural firing and intense pain.
Microglia release many substances that cause pain neurons to become hypersensitive after nerve injury, but their unexpected action on PNNs has a major advantage: specificity. “Usually what perineuronal nets do is they lock plasticity, and they also protect cells,” Khoutorsky said. “So why are these nets only around these pain relay neurons, and not around other cell types [nearby]?” He suspects that it’s because this pain relay point in the spinal cord is so important that these neurons and their connections need extra protection so that their control of pain transmission is strong and reliable. Only something as dramatic as a neural injury can disrupt that stability.
“The beauty of this mechanism is that it is selective for specific cell types,” Khoutorsky said. The substances microglia release to increase neural firing and cause pain after neural injury affect all types of cells in the vicinity, but the PNNs encase only these neurons precisely at the critical relay point in the spinal cord.
Research is underway to better understand this new mechanism of chronic pain. If researchers can develop methods to rebuild PNNs on these neurons after injury, it could provide a new treatment for chronic pain — an urgent need, considering that opiates, the current solution, lose their potency over time and can become addictive or result in a fatal overdose.
What goes on inside neurons is fascinating and important to understand, but neural networks are formed by individual neurons linked together, and here it is the neglected cartilaginous cement in the space between them that is vital.