Science’s Path From Myth to Multiverse
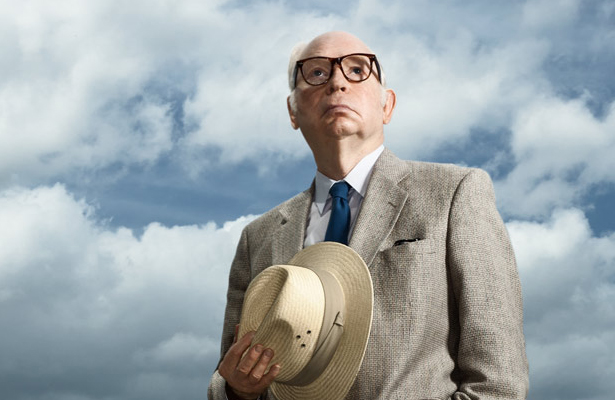
We can think of the history of physics as an attempt to unify the world around us: Gradually, over many centuries, we’ve come to see that seemingly unrelated phenomena are intimately connected. The physicist Steven Weinberg of the University of Texas, Austin, received his Nobel Prize in 1979 for a major breakthrough in that quest — showing how electromagnetism and the weak nuclear force are manifestations of the same underlying theory (he shared the prize with Abdus Salam and Sheldon Glashow). That work became a cornerstone of the Standard Model of particle physics, which describes how the fundamental building blocks of the universe come together to create the world we see.
In his new book To Explain the World: The Discovery of Modern Science, Weinberg examines how modern science was born. By tracing the development of what we now call the “scientific method” — an approach, developed over centuries, that emphasizes experiments and observations rather than reasoning from first principles — he makes the argument that science, unlike other ways of interpreting the world around us, can offer true progress. Through science, our understanding of the world improves over time, building on what has come before. Mistakes can happen, but are eventually corrected. Weinberg spoke with Quanta Magazine about the past and future of physics, the role of philosophy within science, and the startling possibility that the universe we see around us is a tiny sliver of a much larger multiverse. An edited and condensed version of the interview follows.
QUANTA MAGAZINE: As a physicist, how is your perspective on the history of science different from that of a historian?
STEVEN WEINBERG: One difference, of course, is that they know more than I do — at least, in their particular field of specialization. Real historians have a much better grasp of the original sources than I could possibly have. If they’re historians of the ancient world, they’ll be experts in Greek and Latin, which I’m not even remotely knowledgeable about.
But there’s also a difference in attitude. Many historians are strongly opposed to the so-called “Whig interpretation” of history, in which you look at the past and try to pick out the threads that lead to the present. They feel it’s much more important to get into the frame of mind of the people who lived at the time you’re writing about. And they have a point. But I would argue that, when it comes to the history of science, a Whig interpretation is much more justifiable. The reason is that science, unlike, say, politics or religion, is a cumulative branch of knowledge. You can say, not merely as a matter of taste, but with sober judgment, that Newton knew more about the world than Aristotle did, and Einstein knew more than Newton did. There really has been progress. And to trace that progress, it makes sense to look at the science of the past and try to pick out modes of thought that either led to progress, or impeded progress.
Why did you focus on the history of physics and astronomy?
Well, that’s what I know about; that’s where I have some competence. But there’s another reason: It’s in physics and astronomy that science first became “modern.” Actually, it’s physics as applied to astronomy. Newton gave us the modern approach to physics in the late 17th century. Other branches of science became modern only more recently: chemistry in the early 19th century; biology in the mid-19th century, or perhaps the early 20th century. So if you want to understand the discovery of modern science — which is the subtitle of my book — that discovery was made in the context of physics, especially as applied to astronomy.
Theoretical physics is often seen as a quest for unification — we think of Newton, unifying terrestrial and celestial physics, or James Clerk Maxwell, unifying electricity, magnetism, and light. And of course your own work. Where does this quest for unification stand today?
It hasn’t advanced very much, except for the fact that the theories we speculated about in the 1960s have been confirmed by observation. In the theory I developed in 1967 — Abdus Salam developed essentially the same theory, independently, in 1968 — a symmetry-breaking field played a fundamental role, manifest in a particle called the Higgs boson, whose properties we predicted, except for its mass. Now, thanks to experiments performed at CERN, the Higgs has been verified. So we’re on much more solid ground. But we haven’t gone any further. There have been enormous efforts to take further steps, especially in the context of string theory. String theory would unify all of the forces — the strong and weak nuclear forces, and the electromagnetic force, together with gravity. String theory has provided some deep mathematical ideas about how that might work. But we’re far from being able to verify the theory — much further than we were from verifying the electroweak theory 40 years ago.
The Large Hadron Collider (LHC) is scheduled to start up again this year, with twice the power it had during its initial run. What do you hope it’ll find — I’m not sure if “hope” is the right word — when it’s turned on?
The Standard Model is so complex that it would be hard to put it on a T-shirt.
Hope is exactly the right word! It depends on what new particles might have masses in the range that the LHC can probe. There are certainly things to look for. The most obvious thing is the dark-matter particle. We know from astronomy that five-sixths of the matter in the universe is something that doesn’t fit in the Standard Model of particle physics. But we have no idea what its mass is. Astronomers can tell us the total mass of this dark matter, but not the mass carried by each particle. If it’s a conventional dark-matter particle, known as a WIMP — “weakly interacting massive particle” — then the LHC might find it. It depends on how heavy it is, and on how it decays, because you never see the particle itself, you only see the products of its decay.
The LHC might also find signs of supersymmetry, a theory positing that known particles each have a partner particle — but again, we don’t know what the mass of those partner particles would be. And here, there’s an even deeper uncertainty: We don’t know if supersymmetry has anything to do with the real world. There could also be heavier quarks, perhaps even heavier versions of the Higgs particle.
It’s sometimes said that supersymmetry would be a kind of thumbs-up for string theory, which has been impossible to test in any direct way. If the LHC finds no evidence for supersymmetry, what happens to string theory?
Damned if I know! Unfortunately, string theory doesn’t make very specific predictions about physics at the energies that are accessible to us. The kind of energies of the structures that string theory deals with are so high, we’ll probably never be able to reproduce them in the lab. But those energies were common in the very early universe. So by making cosmological observations, we may get a handle on the physics of those incredibly high energies. For example, if the matter-energy density at the time of inflation was of the order of magnitude that is characteristic of string theory, then a great deal of gravitational radiation would have been produced at that time, and it would have left an imprint on the cosmic microwave background. Last year, scientists working with the BICEP2 telescope announced that they had found these gravitational waves; now it seems they were actually measuring interstellar dust. Further observations with the Planck satellite may be able to settle this question. I think that’s one of the most exciting things going on in all of physical science right now.
For theorists, is the ultimate goal a set of equations we could put on a T-shirt?
That’s the aim. The Standard Model is so complex that it would be hard to put it on a T-shirt — though not impossible; you’d just have to write kind of small. Now, it wouldn’t take gravity into account, so it wouldn’t be a “theory of everything.” But it would be a theory of all the other things we study in our physics laboratories. The Standard Model is sufficiently complicated, and has so many arbitrary features, that we know it’s not the final answer. The goal would be to have a much simpler theory with fewer arbitrary features — maybe even none at all — that would fit on a T-shirt. We’re not there yet.
Some physicists suggest that we may have to settle for an array of different theories, perhaps representing different solutions to string theory’s equations. Maybe each solution represents a different universe — part of some larger “multiverse.”
I am not a proponent of the idea that our Big Bang universe is just part of a larger multiverse. It has to be taken seriously as a possibility, though. And it does lead to interesting consequences. For example, it would explain why some constants of nature, particularly the dark energy, have values that seem to be very favorable to the appearance of life. Suppose you have a multiverse in which constants like dark energy vary from one big bang to another. Then, if you ask why it takes the value it does in our Big Bang, you have to take into account that there’s a selection effect: It’s only in big bangs where the dark energy takes a value favorable to the appearance of life that there’s anybody around to ask the question.
You don’t have to verify every prediction to know that a theory is correct.
This is very closely analogous to a question that astronomers have discussed for thousands of years, concerning the Earth and the sun. Why is the sun the distance that it is from us? If it were closer, the Earth would be too hot to harbor life; if it were further away, the Earth would be too cold. Why is it at just the right distance? Most people, like Galen, the Roman physician, thought that it was due to the benevolence of the gods, that it was all arranged for our benefit. A much better answer — the answer we would give today — is that there are billions of planets in our galaxy, and billions of galaxies in the universe. And it’s not surprising that a few of them, out of all those billions, are positioned in a way that’s favorable for life.
But at least we can see some of those other planets. That’s not the case with the universes that are said to make up the multiverse.
It’s not part of the requirement of a successful physical theory that everything it describes be observable, or that all possible predictions of the theory be verifiable. For example, we have a very successful theory of the strong nuclear forces, called quantum chromodynamics [QCD], which is based on the idea that quarks are bound together by forces that increase with distance, so that we will never, even in principle, be able to observe a quark in isolation. All we can observe are other successful predictions of QCD. We can’t actually detect quarks, but it doesn’t matter; we know QCD is correct, because it makes predictions that we can verify.
Similarly, string theory, which predicts a multiverse, can’t be verified by detecting the other parts of the multiverse. But it might make other predictions that can be verified. For example, it may say that in all of the big bangs within the multiverse, certain things will always be true, and those things may be verifiable. It may say that certain symmetries will always be observed, or that they’ll always be broken according to a certain pattern that we can observe. If it made enough predictions like that, then we would say that string theory is correct. And if the theory predicted a multiverse, then we’d say that that’s correct too. You don’t have to verify every prediction to know that a theory is correct.
When we talk about the multiverse, it seems as though physics is brushing up against philosophy. A number of physicists, including Stephen Hawking and Lawrence Krauss, have angered philosophers by describing philosophy as useless. In your new book, it sounds as if you agree with them. Is that right?
I think academic philosophy is helpful only in a negative sense — that is, sometimes physicists get impressed with philosophical ideas, so that it can be helpful to hear from experts that those ideas have been challenged within the philosophical community. One example is positivism, which decrees that you should only talk about things that are directly detectable or observable. I think philosophers themselves have challenged that, and it’s good to know that.
On the other hand, a kind of philosophical discussion does go on among physicists themselves. For example, the discussion we were having earlier about the multiverse raised the issue of what we expect from a scientific theory — when do we reject it as being outside of science; when do we accept it as being confirmed. Those are meta-scientific questions; they’re philosophical questions. The scientists never seem to reach an agreement about those things — like in the case of the multiverse — but then, neither do the professional philosophers.
And sometimes, as with the example of positivism, the work of professional philosophers actually stands in the way of progress. That’s also the case with the approach known as constructivism — the idea that every society’s scientific theories are a social construct, like its political institutions, and have to be understood as coming out of a particular cultural milieu. I don’t know whether you’d call it a philosophical theory or a historical theory, but at any rate, I think that view is wrong, and I also think it could impede the work of science, because it takes away one of science’s great motivations, which is to discover something that, in an absolute sense, divorced from any cultural milieu, is actually true.
You’re 81. Many people would be thinking about retirement, but you’re very active. What are you working on now?
There’s something I’ve been working on for more than a year — maybe it’s just an old man’s obsession, but I’m trying to find an approach to quantum mechanics that makes more sense than existing approaches. I’ve just finished editing the second edition of my book, Lectures on Quantum Mechanics, in which I think I strengthen the argument that none of the existing interpretations of quantum mechanics are entirely satisfactory.
I don’t intend to retire, because I enjoy doing what I’m doing. I enjoy teaching; I enjoy following research; and I enjoy doing a little research on my own. The year before last, before I got onto this quantum mechanics kick, I was writing papers about down-to-earth problems in elementary particle theory; I was also working on cosmology. I hope I go back to that.
This article was reprinted on ScientificAmerican.com.