The First Nuclear Clock Will Test if Fundamental Constants Change
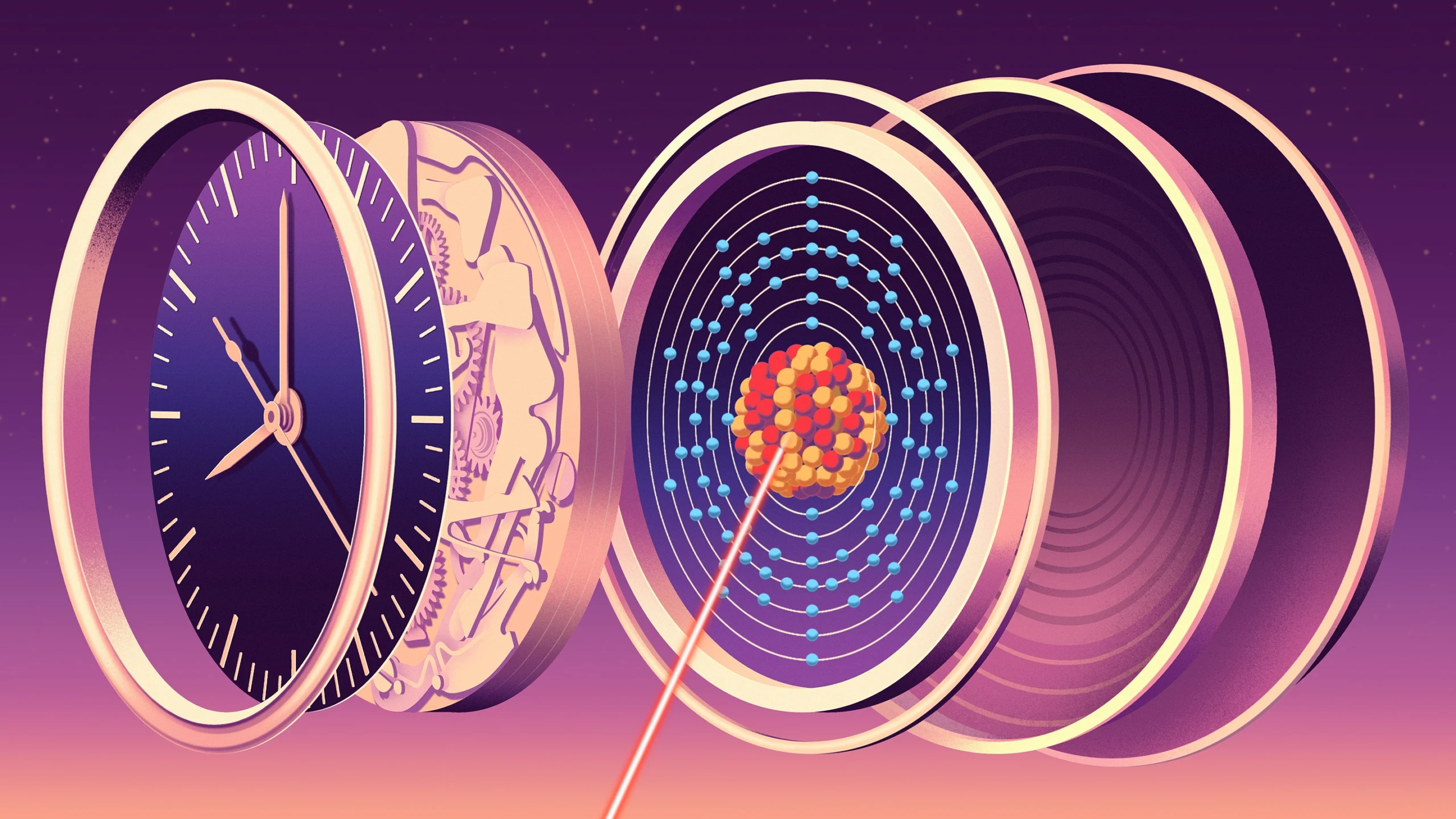
The discovery of a laser-controllable transition in the atomic nucleus of thorium-229 marks the dawn of the “nuclear clock.”
Nash Weerasekera for Quanta Magazine
Introduction
At 11:30 one night in May 2024, a graduate student, Chuankun Zhang, saw a signal that physicists have sought for 50 years. As a peak rose from the static on his monitor at the research institute JILA in Boulder, Colorado, Zhang dropped a screenshot in a group chat with his three lab mates. One by one they hopped out of bed and trickled in. After several sanity checks to make sure that what they were looking at was real — a signal from a thorium-229 nucleus switching between two states, known as the “nuclear clock” transition — the young researchers took a selfie to commemorate the moment. Time stamp: 3:42 a.m.
At their weekly meeting later that morning with their group leader, Jun Ye, builder of the world’s most precise atomic clock, they decided to play it cool. “They were all poker-faced,” Ye said, until Zhang shared a slide displaying the long-sought peak. Tears flooded Ye’s eyes as the group clinked glasses of champagne at 9:30 a.m.
The group’s measurement, reported on September 4, 2024, in the journal Nature, is the third observation of the thorium-229 transition published within the last four months, coming on the heels of results from Germany and California. But the new measurement is millions of times more precise than the others, and it marks the end of a marathon search for the exact laser frequency needed to induce the nuclear clock transition. “This paper is an incredible technical achievement,” said Hannah Williams, a physicist at Durham University in the United Kingdom who was not involved in the work.
More importantly, it launches a new effort: Researchers will now try to use the transition to observe whether the laws of physics vary over time, as predicted by many theories of fundamental physics. Thanks to an apparently accidental, nearly exact cancellation of two of nature’s four forces in the thorium-229 nucleus, the nuclear clock transition is extremely sensitive to changes in these forces. Measuring this thorium-229 transition at different times could therefore reveal any variability in the fundamental constants of physics.
“I see it as the beginning of a beautiful journey,” said Asimina Arvanitaki, a theoretical physicist at the Perimeter Institute for Theoretical Physics in Canada who was also not involved. “Now we’ve measured this freak of nature. But in order to use its freakiness, a lot of work needs to be done.”
Freak of Nature
Scientists realized there’s something special about the isotope thorium-229 back in 1976, when they first studied this byproduct of Cold War nuclear weapons research.
Atoms are ordinarily in what’s called the ground state, in which all the electrons orbit the nucleus in a stable way. But an electron can also absorb energy from the outside world in the form of a photon and become excited, zip about the atom more quickly for a moment, then reemit the photon and return to the ground state. The photon has to have just the right amount — or “quantum” — of energy to excite the electron.
The modern notion of time is actually defined by this process. Scientists use a laser to bathe a cesium atom with photons. Then they vary the laser’s wavelength until its photons each have just the right energy to excite an electron. This ultra-precise wavelength then defines the international standard for a second, which is the time it takes for 9,192,631,770 of those wavelengths to pass a given point in space.
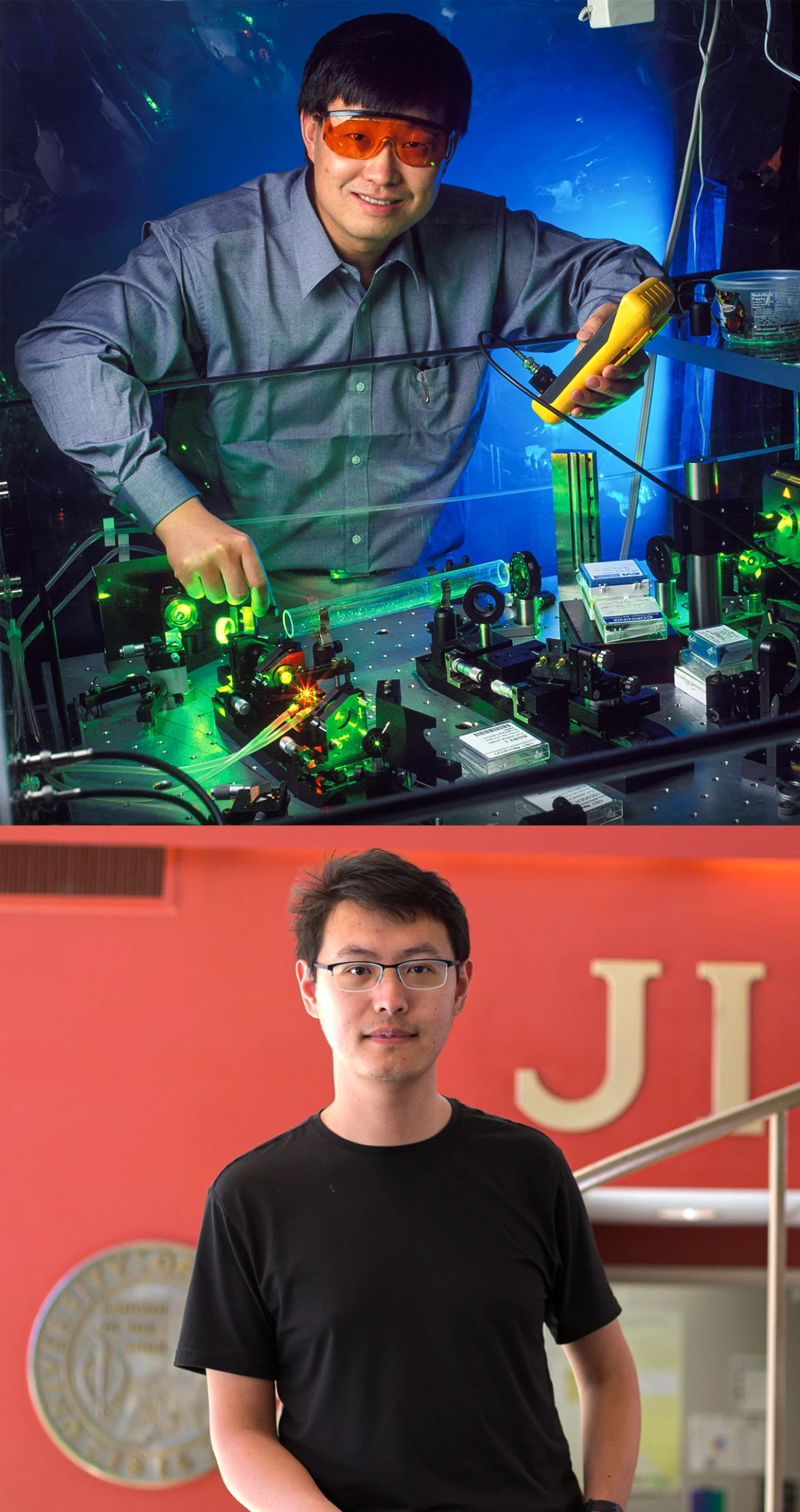
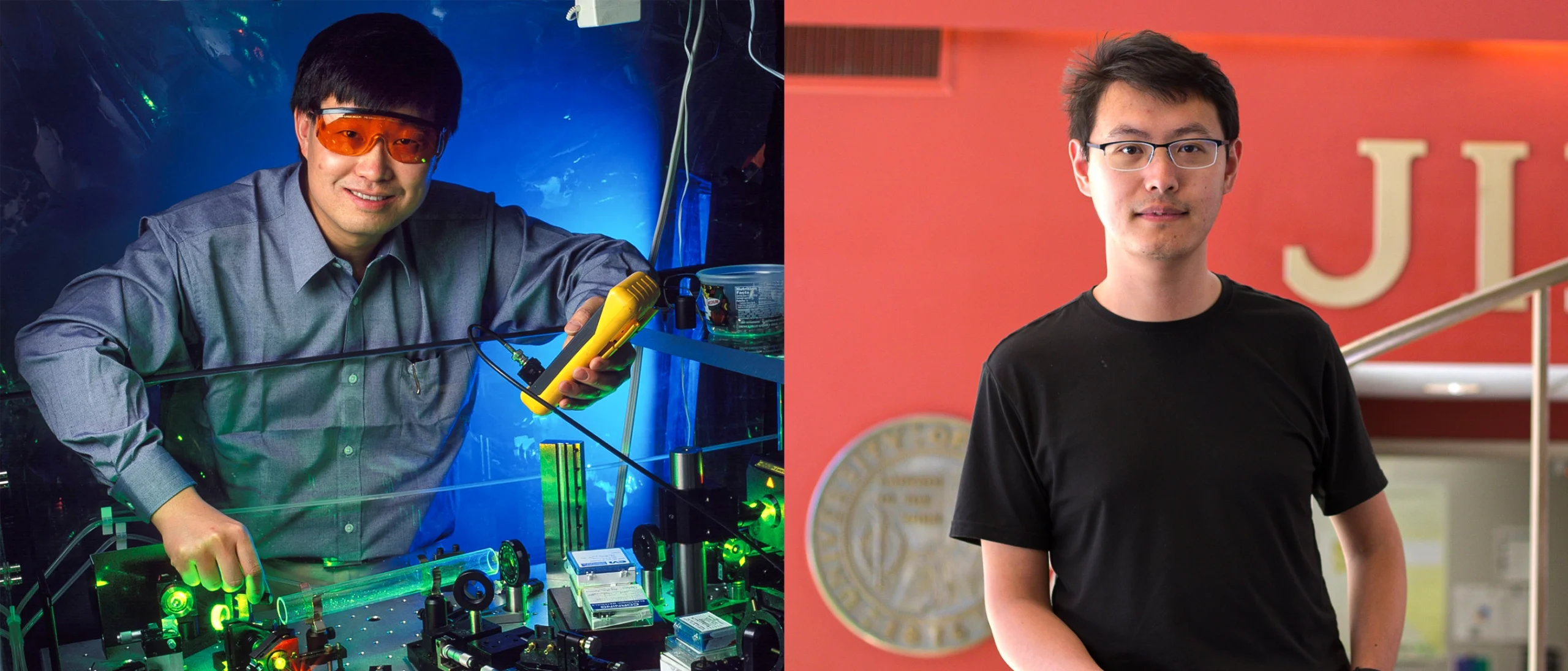
A team in the lab of Jun Ye (top), led by graduate student Chuankun Zhang (bottom), published an ultra-precise measurement of the nuclear clock transition in the journal Nature.
A team in the lab of Jun Ye (left), led by graduate student Chuankun Zhang (right), published an ultra-precise measurement of the nuclear clock transition in the journal Nature.
From left: Geoffrey Wheeler; Kenna Hughes-Castleberry/JILA
Nuclei, the tight balls of neutrons and protons at every atom’s core, also have ground and excited states, in which one of their constituent protons or neutrons absorbs a photon and briefly swirls about more energetically. But these particles are packed much more tightly than electrons, so it takes much more energetic photons — gamma rays — to excite them. Those are much harder to produce in large quantities or with a precise energy.
The thorium-229 nucleus, however, is different.
From the 1950s to the 1970s, the United States produced about two tons of uranium-233, a weapons-grade fissile material that was being investigated as a possible alternative to uranium-235 and plutonium-239 in atomic weapons research. The program was eventually scrapped, leaving only some tanks of radioactive liquid behind. But when the nuclear physicists Larry Kroger and Charles Reich at Idaho National Laboratory studied the radiation emanating from that liquid in 1976, they found indirect evidence that uranium-233’s “daughter” nucleus (the product of its radioactive decay), thorium-229, had a mysterious excited nuclear state that involved far less energy than expected.
Every nucleus lives in a tense tug-of-war between two of nature’s forces. The electromagnetic force between its positively charged protons tries to rip it apart, while the strong force holds the bundle together. Exciting a neutron or proton causes the nucleus to settle into a new, more energetic equilibrium between the two forces.
The Idaho researchers observed that reversing the intrinsic angular momentum, or “spin,” of thorium-229’s outermost neutron seemed to take 10,000 times less energy than a typical nuclear excitation. The neutron’s altered spin slightly changes both the electromagnetic and strong forces, but those changes happen to cancel each other out almost exactly. Consequently, the excited nuclear state barely differs from the ground state. Lots of nuclei have similar spin transitions, but only in thorium-229 is this cancellation so nearly perfect.
“It’s accidental,” said Victor Flambaum, a theoretical physicist at the University of New South Wales in Sydney. “A priori, there is no special reason for thorium. It’s just experimental fact.” But this accident of forces and energy has big consequences.
Clocking the Constants
It took decades for scientists to realize just how special thorium-229 is, and what to do with it.
Kroger and Reich’s 1976 measurement had been imprecise, because it was carried out in the noisy bath of radiation that uranium-233 waste produces. They couldn’t see the actual low-energy photons released when nuclei decayed to the ground state; they only inferred the energy indirectly from the pattern of more powerful gamma radiation emitted by more excited nuclei.
In 1990, Reich and a colleague redid this measurement more carefully and found that the excited state’s energy was even smaller — more than 10 times smaller — than they initially thought. Whereas nuclear transitions often take millions of electron-volts, thorium-229 takes less than 10. This was a true game changer: No other isotope has a nuclear transition in the energy range of conventional lasers, which can deliver the energy to trigger the transition reliably and precisely. “In the whole chart of all the nuclei, it’s the only one,” said Eric Hudson, a physicist at the University of California, Los Angeles.
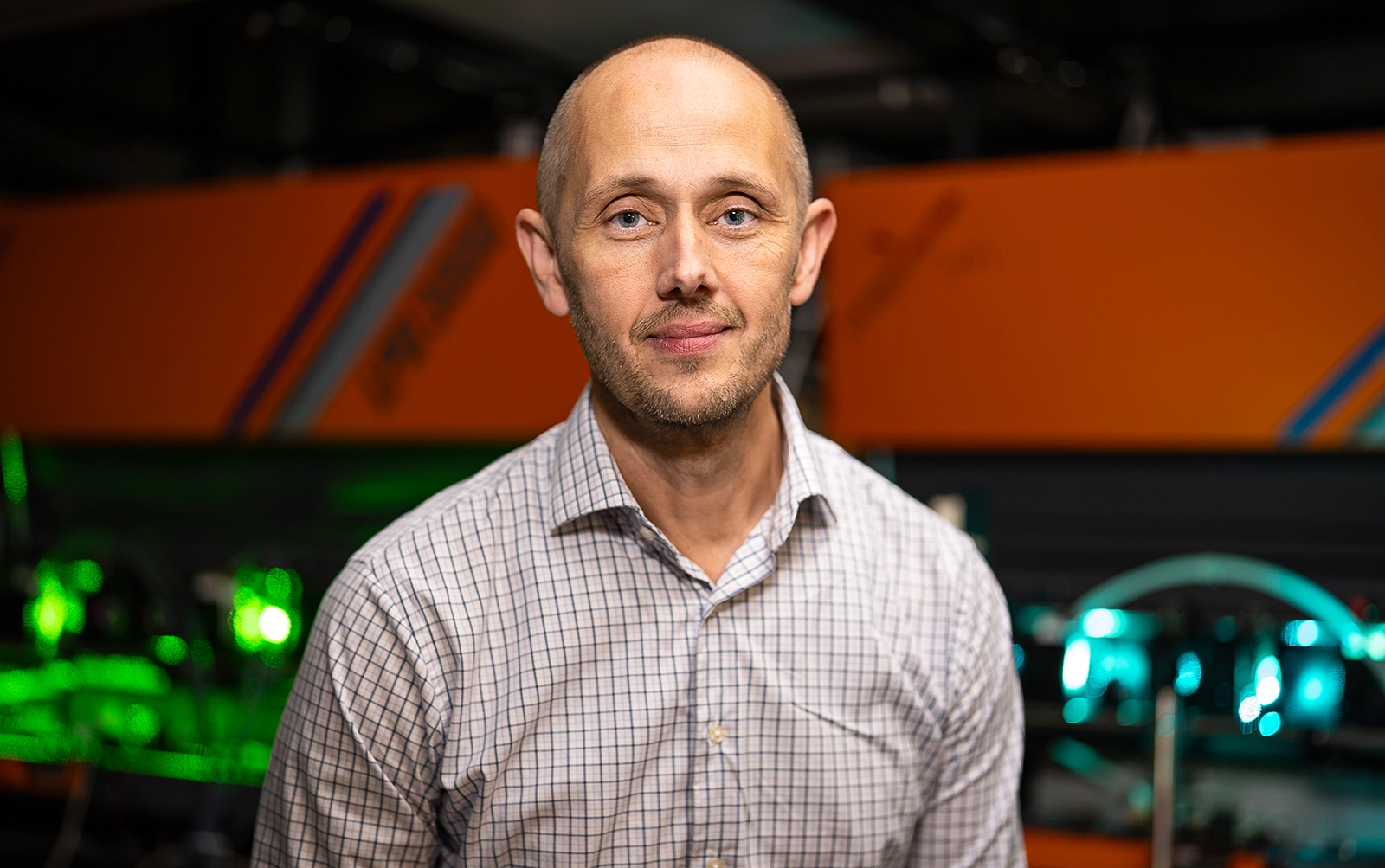
Eric Hudson’s team at the University of California, Los Angeles reported a measurement of the nuclear clock transition over the summer.
David Esquivel/UCLA
If someone could isolate these nuclei from that radioactive environment and match an ultraviolet laser’s energy to that excited state, they could trigger it at will, just as they can with an electron.
The vast majority of the government’s uranium-233 “waste” still sat in guarded rooms at the Idaho and Oak Ridge labs. “Their budget was something like $20 million a year to just sit there and watch the stuff so nobody comes in and steals it,” said Saed Mirzadeh, a radiochemist who worked for 31 years at Oak Ridge. “They’d just sit there and smoke their cigarettes with guns around their necks.”
In 1994, Mirzadeh, who knew about the Idaho team’s work, convinced the lab to give him access to the languishing vats of dangerous liquid. He developed a method to separate the uranium atoms that had already decayed into thorium-229 from those that hadn’t. “The first time we actually did it, there were guards with machine guns outside the lab,” he said. Most of the world’s existing stock of thorium-229, he noted, comes from his efforts.
Ideas began to arise for how to utilize such a unique nucleus. In 2003, Ekkehard Peik and Christian Tamm at the Federal Physical and Technical Institute (PTB), Germany’s metrology institute, proposed using it to build a nuclear clock. Since nuclei are shielded from the outside world by their clouds of electrons, they realized, a clock based on thorium-229 atoms would be immune to much of the background interference that plagued the best atomic clocks at the time.
Then Flambaum showed that such a sensitive, isolated clock could be used to test the constancy of nature itself.
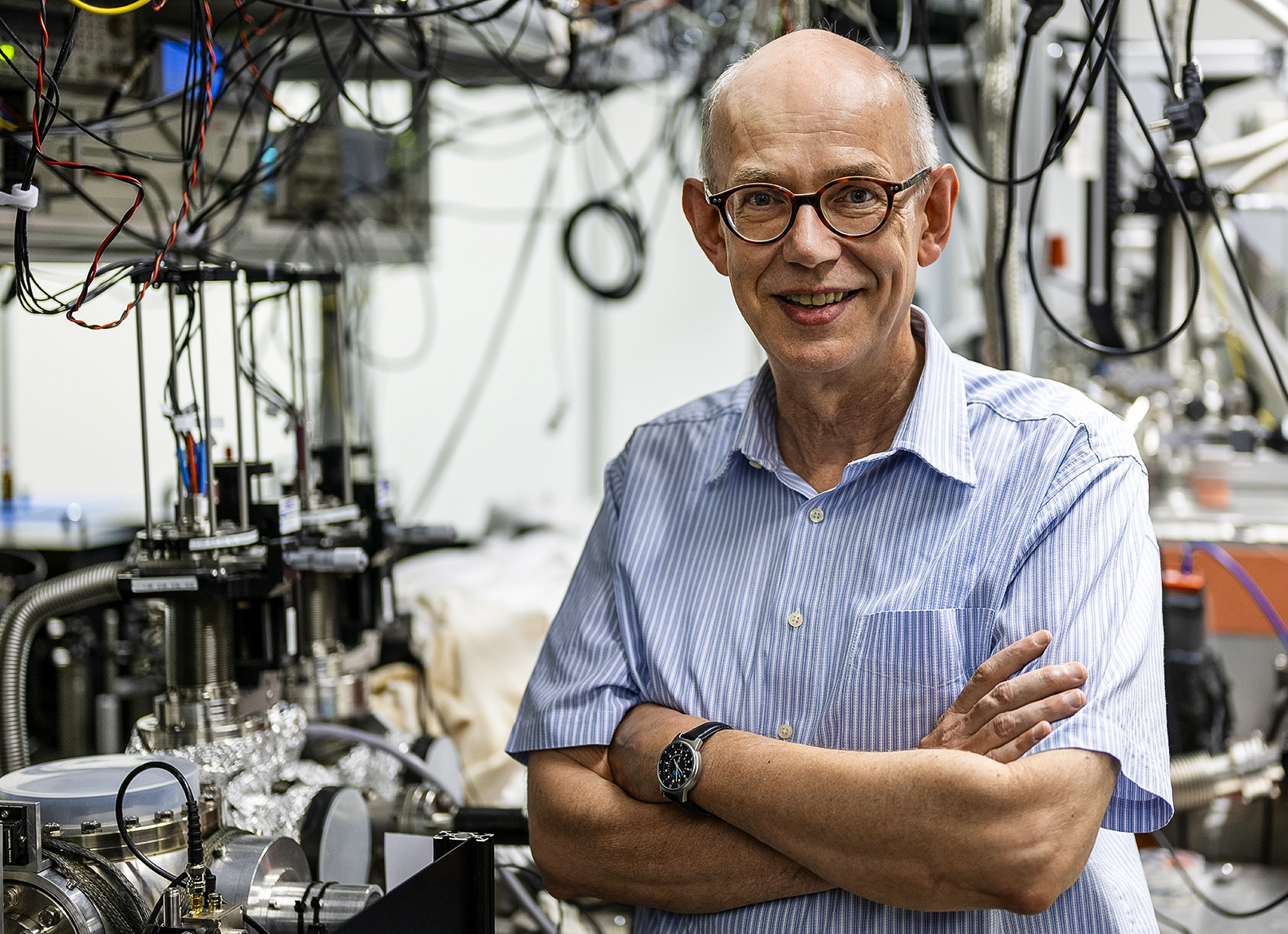
Ekkehard Peik at the Federal Physical and Technical Institute in Germany and his collaborators were the first to excite the nuclear clock transition with a laser earlier this year.
Physikalisch-Technische Bundesanstalt
Physicists have developed equations to characterize the forces that bind the universe, and these equations are fitted with some 26 numbers called fundamental constants. These numbers, such as the speed of light or the gravitational constant, define how everything works in our universe. But lots of physicists think the numbers might not actually be constant.
Theoretical ideas like string theory that try to build a deeper, more complete understanding of where forces come from often predict that these numbers, even the speed of light, change ever so slightly over time. In other words, the constants may result from underlying phenomena or processes that are themselves dynamic. This is also predicted by one of the most popular theories of dark matter, the invisible substance that floats in and around galaxies. If dark matter is made up of wavelike particles called axions, then the varying density of axions from place to place should cause the strength of some of the forces to wiggle up and down.
These small tweaks to nature’s laws could slightly disrupt the delicate balancing act that takes place inside every atom’s nucleus, altering the energies of its states. The energies of nuclear states come from adding and subtracting the huge electromagnetic and strong forces acting on all the protons and neutrons. Even a relatively small change in the strength of one of these forces would result in a substantial shift in energy. The shift would be especially noticeable when applied to the thorium-229 transition’s remarkably tiny energy.
Over the 2000s and 2010s, several teams entered the race to build the first nuclear clock. To win, they needed to figure out the exact energy a laser would need to excite the nuclear state in question, now called the nuclear clock transition.
Photo Finish
The existing estimate of the energy required for the nuclear clock transition was a thousand times less precise than the wavelengths of the lasers that researchers were trying to probe it with. So there were thousands of laser wavelengths to rule out. After tuning a laser to one of these wavelengths, researchers had to trap a few thorium-229 atoms, hit them with the laser, then wait for photons showing they’d excited the state. This process of elimination was simply going to take too long.
Following Hudson’s lead, groups began building solid crystal compounds with the thorium embedded inside — an approach mentioned in Peik and Tamm’s original proposal. The crystals can hold quadrillions of atoms instead of just a few, so a laser could rule out wavelengths at a rapid clip.
A breakthrough last year at CERN kicked the race into overdrive. As in the older Idaho studies, the CERN team produced excited thorium-229 through radioactive decay, then looked at the photons coming out. But they found a way to do so in a much quieter environment, which enabled them to directly measure the faint rays of ultraviolet light coming from the nuclear clock transition and put a tighter estimate on the transition energy.
The CERN team’s updated estimate narrowed the wavelength hunters’ search from an entire forest to a small copse of trees, which they immediately began scouring. In April of this year, a European team became the first to report that they had probed the state with a laser. Peik contributed his laser expertise, and the collaboration made use of a crystal-growing powerhouse built by the physicist Thorsten Schumm at the University of Vienna.
Hudson’s group was right on their heels — a paper reporting their discovery ran in Physical Review Letters in July.
Ye’s group at JILA had also obtained one of Schumm’s crystals and was racing to excite the thorium-229 transition as well. For years, the group has been using its clock-building acumen to engineer a special ultraviolet laser with the sole purpose of turning thorium-229 into a nuclear clock. The laser allows Ye and his group to test many wavelengths at once to close in on any transition he seeks. His team’s new paper caps this trio of parallel discoveries with what will likely be the most precise measurement of the state’s energy for years to come.
“These results have all come out in a very short period of time,” Williams said, “so that is very exciting as to what they’re going to do next.”
The result starts the clock on thorium’s test of nature’s forces. “Now the fun starts,” Hudson said, excited to put the new tool to use studying fundamental constants. “We can actually do this stuff.”
The thorium nuclear state’s energy is far more sensitive to variations in the fundamental constants than that of any atomic state. But scientists will need to improve the precision of their measurements even further to notice changes more subtle than those already ruled out by conventional atomic clocks. Currently, Ye can measure the nuclear clock transition with a precision of one part in a trillion, but possible variations would be as small as one part in 10 trillion. “It’s many years down the road,” he said.
Eventually, though, some old Cold War byproducts could yield the first evidence for deeper, still undiscovered physics that underlies the universe we see. “We call them constants, but why?” Hudson asked. “Nothing is ever that simple when you zoom in and look at it.”