Will Better Superconductors Transform the World?
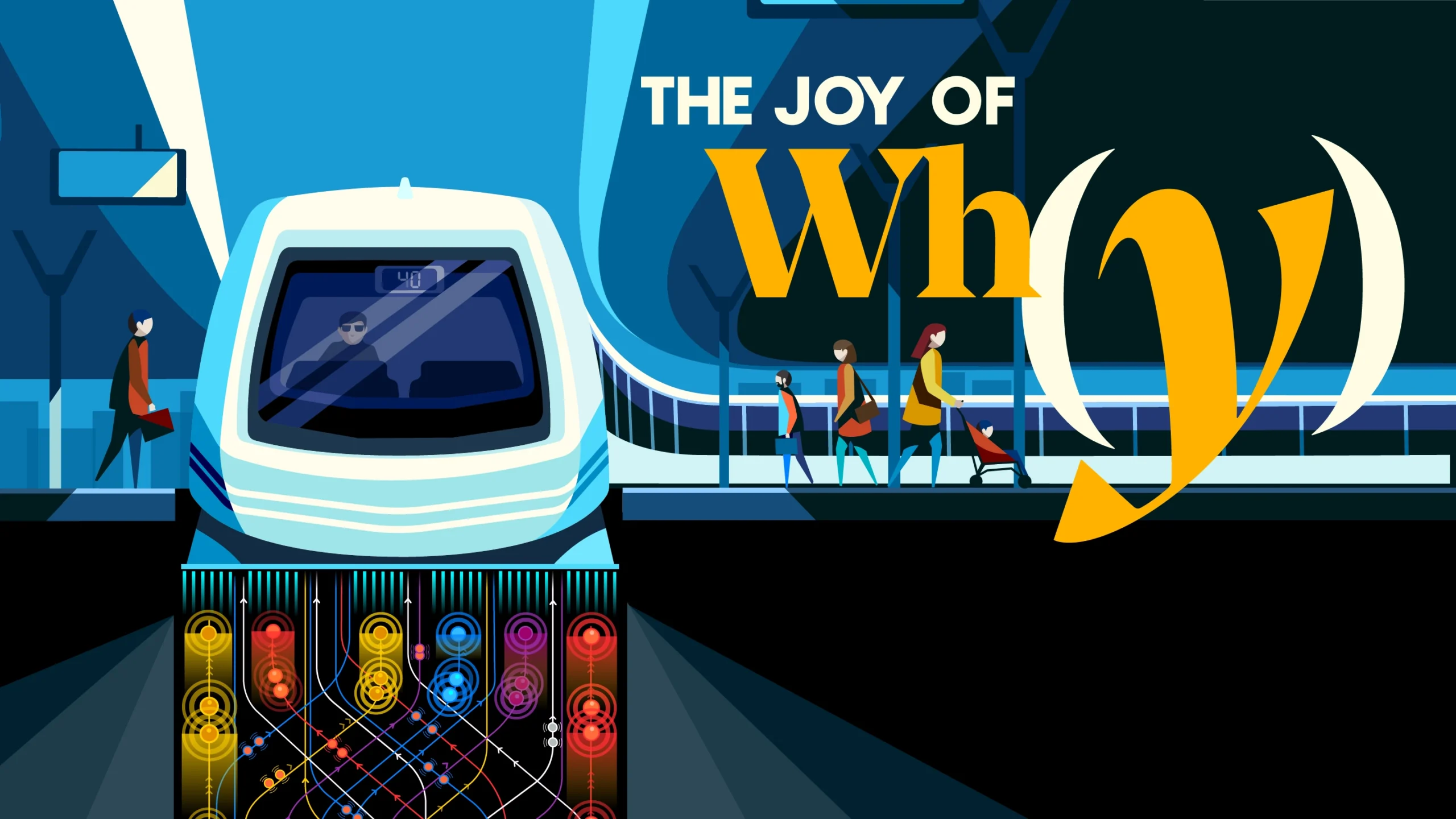
Peter Greenwood for Quanta Magazine
Introduction
If superconductors — materials that conduct electricity without any resistance — worked at temperatures and pressures close to what we would consider normal, they would be world-changing. They could dramatically amplify power grids, levitate high-speed trains and enable more affordable medical technologies. For more than a century, physicists have tinkered with different compounds and environmental conditions in pursuit of this elusive property, but while success has sometimes been claimed, the reports were always debunked or withdrawn. What makes this challenge so tricky?
In this episode, Siddharth Shanker Saxena, a condensed-matter physicist at the University of Cambridge, gives co-host Janna Levin the details about why high-temperature superconductors remain so stubbornly out of reach..
Listen on Apple Podcasts, Spotify, TuneIn or your favorite podcasting app, or you can stream it from Quanta.
Transcript
[Theme plays]
JANNA LEVIN: On April 8th, 1911, a Dutch scientist made a chilling discovery. Using a carefully engineered instrument filled with liquid helium, physicist Heike Kamerlingh Onnes delicately lowered the temperature of mercury closer and closer to absolute zero. Suddenly, at an unimaginably cold negative 452 Fahrenheit, the supercooled mercury conducted electricity with perfect efficiency and no energy loss to heat. It was the first superconductor.
I’m Janna Levin, and this is “The Joy of Why,” a podcast from Quanta Magazine, where I take turns with my co-host, Steve Strogatz, exploring the biggest questions in math and science today.
The discovery of superconductivity would win Kamerlingh Onnes the 1913 Nobel Prize in Physics.
[Theme ends]
But more importantly, it marked the start of an unresolved quest for material that maintains perfect conductivity at room temperature. A quest that’s recently seen many claims put forward and then retracted. Today, we ask physicist Siddharth Shanker Saxena, known to his friends as Montu: What makes room-temperature superconductivity so elusive and how might its discovery reshape society?
Montu is a principal research associate in the Cavendish Laboratory at the University of Cambridge, researching superconductors, magnets, graphite and renewable energy applications. He also teaches at Cambridge’s Center for Development Studies, and chairs the Cambridge Central Asia Forum. Welcome to the show, Montu.
SIDDHARTH SHANKER SAXENA: Thank you, Janna. Excellent intro.
LEVIN: Thank you.
SAXENA: And I think a very fitting thing to say: “chilling discovery.”
LEVIN: Yes, our script writers like puns. Before we get into the absolutely amazing and unforeseen phenomena of superconductivity, I want to talk about regular conductivity. We have fundamental elements just on our periodic table, things that are made in the universe, that are conductors, and we call them metals. Can you tell us just very briefly the basics of conductivity?
SAXENA: Sure. So there are different ways of understanding conductivity. Conductivity of water, conductivity of heat, conductivity of electricity. And these properties of elements, it’s one side commonplace, and another side extremely mystical.
Like, when you walk into a room, you flick a switch, and something happens. We can say a circuit is completed. The current starts to flow. It can only flow in a conductor. It’s a conduit through which things can flow. And if it’s not, it stops flow, it’s an insulator.
So you flick that switch, and you allow the electrons to flow all the way to your light bulb, your computer, your fan, or whatever else that is, and it starts to work. And that is conductivity and the material property needed for that to happen is metallicity. Something has to be metallic for it to have basic conducting properties. Of course, there are various caveats and that hopefully we’ll chat about as we go forward.
LEVIN: Yeah, so basically these elements in the periodic table are all lined up on one side because they allow their electrons to do what you’re describing. To run freely through the material, carrying energy with them. And insulators hang on to their electrons and don’t allow it to happen. I use a cloth to hold my kitchen pan…
SAXENA: Yes.
LEVIN: But the pan itself, I rely on allowing the conductivity. Now, how is superconductivity so much different from this naturally occurring phenomenon?
SAXENA: First of all, we often think electrons can freely move in a conductor, but they don’t really move freely. That is the reason why you have your electricity bill. When electrons travel through the metal, they fight their way through other electrons and other defects and so on, and lose energy as they move forward. And that loss is what we pay for. And the process that is functionalized when this is happening is limited by the amount of power or current or number of electrons we put into the conduit.
While superconductivity is literally the superpower of those electrons. They can travel this time freely without being inhibited by other electrons or defects and so on. So once you get the ball rolling, they continue to go until the system cannot sustain that property.
LEVIN: It’s quite amazing, because as you say, energy is money. And so it costs energy every time you waste some of your electricity into heating up your charger, or your phone gets hot, or your computer needs to cool off. That’s all loss. And superconductivity doesn’t have these losses. That’s quite miraculous. Now, what are some examples of superconductors that are actually in application now?
SAXENA: I think the most prominent one that affects our daily — hopefully not our daily lives, but we encounter it — is a CAT scan or MRI scanner. That’s made of a superconducting coil, which is used to generate magnetic fields. And it’s a primary well-being tool that we have.
Perhaps a more fantastic one is the new era of transport. The maglev trains, for example. Levitating trains that go at speeds similar to aircraft.
There are multiple other smaller aspects. Superconductors allow for us to develop very sensitive devices, which can pick up signals which are minute, which would otherwise not be possible to pick up. And they can be applied from anywhere, in IT or healthcare or mining applications or various other things like that.
LEVIN: Now, why can’t I have these fantastic gadgets in my home and save on my energy bills?
SAXENA: That’s literally a million-dollar question, or multimillion-dollar question.
[Both laugh]
SAXENA: And two interesting aspects of this. One is the fact that superconductivity itself, as you started by telling us in the very beginning of the podcast, was when that liquid-filled special device used by Kamerlingh Onnes was used to cool down mercury.
And similarly, we have to cool down most of these metals to a superconducting state. So basically you start with a normal metal, where electrons are fighting thermal barriers, but then you take it through a transition at which it starts to work in the super way. So now, for us to have that property, we need that special device to keep superconductivity maintained at those temperatures. And that’s what has inhibited its general use.
And that is in itself a very interesting problem that, as human beings, we have conquered the heat. We sit in a car, we turn the switch on, we put a newborn baby a foot away from thousands of degrees of heat and not even think about it. At the same time, we don’t have the same kind of control yet over cooling. Superconductivity happens at lower temperatures. However, our ways of cooling still need developing.
LEVIN: Right. So now, after Kamerlingh Onnes’ initial discovery of mercury’s superconductive properties, where did the research go? Did it immediately go to “let’s try to do this at warmer temperatures”? Because, as you said, he had to supercool it to a staggeringly cold temperature to observe this phenomenon.
SAXENA: So, if you look at the history of the development of superconductivity, we’ll find two approaches. And that happens with most physical phenomena which is discovered. The instinct of a physicist, or chemist or basic scientist, is to understand why is it happening at all? Just imagine it was happening in that era when it happened. This was the first time it’s ever been seen. One wasn’t thinking necessarily about using it or controlling it, just to understand what it is. Why is it happening at all? Or is it even true? Can we find other examples of it? Is it just a flaw in the measurement?
The early period went on establishing the phenomenon itself. Understanding its parameters. When it happens, where it happens, how it can happen. And then the next step: Can it happen in things other than mercury? And that’s when the juices start to flow. You start to think we have different materials in which this can happen. Can this happen in alloys? Can it happen in compounds? And as that happens, you start to talk to engineers, you start to talk to people in other fields, and their input is what make you realize that it can possibly be used for other things. And the two efforts started to go in parallel but separate ways: the application of superconductivity versus understanding of superconductivity.
And there’s another very interesting lever here which we haven’t mentioned. It’s not only temperature, it’s also pressure. In fact, room-temperature superconductivity is already discovered. It’s not something that’s elusive anymore, except that it happens at very high pressures. Mikhail Eremets and colleagues in 2015 already produced near-room temperature superconductivity. And now there are various examples of it.
LEVIN: Now let’s talk about why it happens, because it’s extremely different from the ordinary conductivity of the naturally occurring elements. You might imagine if I supercooled something that the electrons’ motions could be inhibited, and that actually conductivity would therefore drop to zero. And so this is really a very different and surprising phenomenon. Can you talk us through the Nobel Prize-winning work of John Bardeen, Leon Cooper and John Schrieffer and their theory of superconductivity?
SAXENA: That was a landmark moment, not just in understanding superconductivity, but understanding quantum phenomena altogether. So BCS theory: The basic essence of their theory is that they were able to explain that the electrons don’t travel alone like they do in a metal. They form a coherent state, what we call the Cooper pair. The two electrons come together and, if you want to use an analogy, they are the bully on the street. They’re able to push everyone else away so they can move effortlessly in this maze of other electrons and so on.
The formation of the Cooper pair was something that was only describable and conceptually tangible through the work of Bardeen, Cooper and Schrieffer in the 1950s. And, in fact, Cooper was the one who understands that when two electrons are able to come together in this way, they form a coherent state. It’s a lot of electrons which become coherent, but they can be described as a set of pairs, or a condensate that forms.
LEVIN: So fascinating because electrons, of course, if you’re in the quantum world or you’ve studied quantum theory, are notorious for not wanting to pair up.
SAXENA: Yep.
LEVIN: And the Pauli exclusion principle famously says that there’s, in fact, a quantum pressure associated with trying to jam electrons together. They don’t like it.
So this phenomenon that they discovered is extremely counterintuitive: the collaboration with the lattice of ions left behind when the electrons start to move away. When they pair up, now they have the opposite phenomenon where they want to bunch together. So you don’t just get one pair. You now have an encouraging accumulation of these electrons and hence this runaway phenomenon of superconductivity. It’s absolutely counterintuitive and fascinating. And that theory has held up well over the years?
SAXENA: Yeah. One picture that the theory builds in your mind, is that of a lattice and the vibrations of lattice, what we call phonons. And so you can think in terms of, if this boat or ship is moving in water and it creates a wake behind it, while it looks like it’s pushing the water back while moving forward, it creates that small wake where things can get trapped, which is attractive potential.
So it held up quite well for a vast majority of superconductors. And one of the important reasons for that is that the electron has the other property, the spin. And spin is what gives it magnetism. So everything we have discussed now is the charge of an electron. And so while we can talk of electron and its Pauli exclusion principle, we now turn to Bohr and spin, and talk about the spin itself and how opposite spin electrons can attract each other. And a Cooper pair has a spin-up/spin-down configuration in the BCS theory description.
LEVIN: Now why does it require — so far, hopefully not forever — this supercooling?
SAXENA: So, the main barrier that one has to overcome is the thermal one. So you can think about how the heat inhibits that coherence to form. It keeps rattling things before they can come into a coherent state. So we need to get to the temperature in which the electrons are interacting with the other electrons rather than other vibrations.
So two things. We have to have a cooperation between the electrons themselves, and the cooperation between those electrons and the lattice. And this lattice is driven by heat. And the lattice continues to be energetically unfavorable till you get to the temperature at which it starts to become cooperative. So we need the thermal side to decrease for the quantum state to form.
LEVIN: So that suggests that achieving room-temperature superconductivity is going to be hard. And as you said, we’ve seen it only under equally extreme conditions of high pressures. Do you think it’s hopeless, our attempts to achieve room-temperature superconductivity without the high pressures?
SAXENA: Can we hold a cube of ice over open flame and it doesn’t melt? When we talk about room-temperature superconductivity, that’s what we’re trying to achieve here in terms of an analogy. And so if that happens, usefulness aside, if can you hold ice cube over fire, it’s just shockingly amazing and mind-blowing.
On the other hand, there are ways in which we can protect the state because we know that when we chemically make compounds, when we change atoms, we change the internal pressure in a material. We increase or decrease the pressure between bonds, between elements, and that changes the properties. So by playing with the material, we are able to create the same conditions as a pressure does.
LEVIN: We’ll be right back.
[Break]
LEVIN: Welcome back to “The Joy of Why.”
So people have really been investigating synthetic materials as a way to make a big breakthrough. Now there have also been a number of very high-profile declarations of success, published in journals as prestigious as Nature, of new materials or synthetic materials that show room-temperature superconductivity. But then they were retracted. So what’s going on with these kind of controversial claims?
SAXENA: Yes, a couple of things to say about that. The first one, I want to reiterate that it’s not the room-temperature superconductivity which is in question. The claims are about ambient pressure or near-ambient pressure, not about room temperature at all. The question is, can it be achieved in ambient conditions?
So first of all, these high-pressure experiments are extremely difficult. I am a high-pressure scientist myself. So I know the difficulty and the challenge that comes with it. At the same time, it is the most productive in this area. And combined with the difficulty of experimental endeavor, and the promise it has for applicability, we feel that people have been rushing to give big answers. And the whole sociology of academic endeavor falling in sync with this very difficult to achieve but very promising area, has produced very interesting, and in many ways destructive, tendencies. I would say that the vast majority of superconductivity researchers are very careful.
There is a very fun word. We call them USOs. So, periodically, just like what happens with UFOs, all of a sudden, somebody declares there’s big news for a flash, and then it’s gone. Many journals have gone into the commercial publishing world rather than academic publishing. So it’s a nexus of several things which have led to these powerful claims.
But as a scientist, it just whets my appetite, rather than discourage. What I am worried about is that the public interest, and thus political interest, in this wonderful phenomenon — extremely unusual, extremely promising — could get damaged if we rely on those USOs.
LEVIN: So can you give us a little bit of intuition briefly about what these synthetic materials are, how they’re arranged out of what we consider to be ordinary atoms? Are they sheets of different fundamental elements? How are they constructed?
SAXENA: Sure. They’re like houses, they come in different shapes and sizes. And similar to houses, they have different who-can-live-in-it, and how comfortable they are. Superconductors actually come in all shapes and forms. So they can come as cubic material. So think of a structure of a rock salt, you can have that cubic material that can also be superconducting. Even gold, you can look at that.
But then you can have graphite, for instance, layers of carbon [inaudible], but also other materials, and they are very weakly bound together. So what makes graphite interesting for scientists, the reason we can write with pencil, is that those layers just fall apart. They just come onto the paper. We can write with it.
That means we can put other things in between, and that can change interactions between the sheets themselves and also between what we put inside those sheets. So these are so-called 2D materials. Before the advent of the recent room temperature, high-pressure superconductors, there was a whole family called high-TC superconductors. And these were mostly two-dimensional materials. And two-dimensionality, until today, has played a very important role in finding superconductivity.
The analogy that I gave you earlier: the boat is moving, and that there’s a small wake which can trap things in it. But if you imagine that all starts of wobble, i.e., 3D. You increase the dimensionality of your motion. Then it becomes less likely that things can get attracted and trapped and become coherent.
You can have another simpler analogy, that if you take a vat of treacle or honey and you throw a pebble in it, it’ll deform. But since it’s viscous, it cannot propagate. So it starts to retract and everything near it can get attracted and coherently bound. But if you take the same thing and start to wobble it in three dimensions, it’s less likely to happen.
So two-dimensionality has played a very important role. And we don’t know if this is true for these high-pressure phases yet. They look more to be 3D. One of the avenues of research, is can we achieve that kind of condition in 2D materials? And that’s where most likely we’re going to see zero pressure room temp superconductivity. It’s likely to come from lower dimensional materials.
LEVIN: A lot of the scientists that I most admire are unafraid of failure — their curiosity outweighs their fear of failure. You mentioned that you’re in this very challenging area of this high-pressure superconductivity. Give us a little glimpse into your process. What is a working day like in your laboratory?
SAXENA: So my high-pressure lab, it’s also a low-temperature lab. High pressures, because it’s the most difficult part of the process, it gets highlighted. In my lab, first thing that happens is making the material, or working with someone who makes the material. And identifying that a material is what it is.
See, this is where the drama is. Even the discussion that we just had about the previous cases, which were overblown, we don’t fully agree because we don’t know what the materials are. So in my lab, we spend most of our time, sometimes more than a year even, trying to hone down on the material is what it is. And that’s where the resilience of the researchers, the Ph.D. students and myself comes in. Because it could turn out that, after a year, this is not something we want to measure anymore. And we look for new systems all the time in which this thing can happen.
And then, we try to cut the sample down to size, literally. Because pressure requires a very small volume. So, a few tens of microns thickness, and a hundred-micron width, and so on, is where the highest pressures experiments happen. So then it comes down to attaching those famous electrodes to the samples, and then trying to put them in the pressure cells. And then you come to the cryostat and you chill it — literally the opposite of watching water boil. When you make a material chemically, it has certain set of atoms arranged in a particular way, and certain properties. So why pressure is a really important tool is that when you compress something uniformly — hydrostatically, in technical words — you change those atomic distances.
And so effectively at each pressure, you have a new material. And at each pressure point, you can then measure all those properties. The nice thing about this way of searching is that you can have very fine steps. You can increase the pressure, you can release the pressure, and you can keep searching for new and new states.
LEVIN: Would it be fair to say that you’re therefore, as you increase the pressure, looking at phase transitions?
SAXENA: We are looking at both. So we’re looking at change and uniform change in properties, and hoping for that very drastic change, and that will be the phase transition, yes.
LEVIN: If one of these USOs were to become a bona fide reproducible synthetic material that shows superconductivity at ordinary conventional conditions that you can find in someone’s apartment, this would doubtless be incredibly lucrative. And that’s because it’s going to have some very serious implications socially. Can you talk me through some of the societal ramifications of succeeding here?
SAXENA: It may sound biased coming from me, being a superconductivity researcher, but it’s obvious that the ramifications of superconductivity being available in ambient conditions are going to be transformative for all things around us. People keep talking about AI all the time. That’s nothing. Here we are talking about energy efficiencies, which are going to transform how we travel, how we communicate. We’re talking about data farms. We’re talking about power grids. We’re talking about ships, planes, everything. Just like all of those things have a conductor in it, it’ll have a superconductor in it.
LEVIN: Presumably, they’re not going to immediately be available freely across the globe. Presumably, there will be some countries that have faster access because of their investment?
SAXENA: That is absolutely correct. The infrastructure, both industrial and scientific, meaning laboratory and the manufacturing, certainly is available only in the global north. And there is obviously a great potential for it in some of the middle-income countries. But that still leaves a huge part of globe out, which cannot produce or sustain production of these kind of materials.
They tend to be in geographies of Eurasia, Australia and some other parts of the globe only. But I would say that it’s not only about where they occur. For example, South Korea, which has zero iron ore deposits, but is one of the biggest ship makers and steelmakers. So it’s more about how you’re plugged into a system, and supply and value chain. It’s not only about having natural resources. And then you have countries of central Asia having vast resources, but they don’t produce any of them in the way they can be used. So that it’s more than just having it. The discovery itself is not going to do it.
LEVIN: Right, we have to remind ourselves that we really are in this together. We have to get along to make the future work. You clearly believe that superconductivity is one day possible at more ordinary conditions. Do you think we’re close?
SAXENA: One way of talking about this: Does a UFO sighting mean that we’re close to finding other aliens? Is it a symptom of that or not? One can think in that way. But from within the field, our progress has been absolutely impressive in the last 20 years. And that has to do with our ability to make materials, and the kind of experiments we can do now using high pressure and high fields and other conditions. So the stage is now set better than ever before to be able to find these materials, or we have found these materials, so how to engineer these to be in ambient conditions.
LEVIN: Fascinating. Now, you have a background not only in physics but also anthropology and history. How do these other subjects inform your both scientific approach and your bigger picture?
SAXENA: I often describe myself as undisciplined because I’m not bound by any particular discipline. I’ve been lucky to have had the chance to study, interact and teach and work in all these areas. But fundamentally, what I’m looking at is the same thing.
For instance, if you have a bottle of water, if you hold that bottle in your hand, reflect for a second that the hand, the bottle and the water are all made of exactly the same elements. But these three things have nothing in common, absolutely nothing. Live, dead, transparent, translucent, liquid, solid, whatever you call it, there’s nothing in common. And this is where we come in, the modern-day scientists, to say: It’s not only about what things are made of. It’s a question of how things interact. And understanding the interaction gives rise to new properties. And they can be quite counterintuitive, but we must find probes to measure and understand those properties. But also find parameters to tune them, like pressure, field temperature and so on.
Human beings are like those particles. And just imagine the pressure-temperature axis in which a human being can survive. It’s extremely narrow. And yet, our interactions with each other and our interactions with the environment produce entirely different cultures, languages, ways of thinking, and science itself.
LEVIN: There’s a question we here at “The Joy of Why” like to ask our guests, and that is, what about your research brings you joy?
[Theme plays]
SAXENA: The discovery. And doing it with the team, with people, the colleagues. That moment of togetherness when we find something together. Discovering something alone is not something that I have found fun. I found that being part of a group who are working towards some aim, going through trials and tribulations, and then the discovery happens. And it’s a reward that’s shared, without having to slice it up.
LEVIN: No, it’s beautiful. At the end of the day, science is a human endeavor. We’ve been speaking with physicist Siddharth Shankar Saxena, that’s Montu to us, on superconductors and their potential to change the world. Thanks so much for joining us, Montu.
SAXENA: Thank you. It’s been a pleasure.
LEVIN: Pleasure to have you here.
“The Joy of Why” is a podcast from Quanta Magazine, an editorially independent publication supported by the Simons Foundation. Funding decisions by the Simons Foundation have no influence on the selection of topics, guests or other editorial decisions in this podcast or in Quanta Magazine.
“The Joy of Why” is produced by PRX Productions; the production team is Caitlin Faulds, Livia Brock, Genevieve Sponsler and Merritt Jacob. The executive producer of PRX Productions is Jocelyn Gonzales. Morgan Church and Edwin Ochoa provided additional assistance.
From Quanta Magazine, John Rennie and Thomas Lin provided editorial guidance, with support from Matt Carlstrom, Samuel Velasco, Nona Griffin, Arleen Santana and Madison Goldberg.
Our theme music is from APM Music. Julian Lin came up with the podcast name. The episode art is by Peter Greenwood and our logo is by Jaki King and Kristina Armitage. Special thanks to the Columbia Journalism School and Burt Odom-Reed at the Cornell Broadcast Studios
I’m your host, Janna Levin. If you have any questions or comments for us, please email us at [email protected]. Thanks for listening.