A Newfound Source of Cellular Order in the Chemistry of Life
Introduction
Imagine packing all the people in the world into the Great Salt Lake in Utah — all of us jammed shoulder to shoulder, yet also charging past one another at insanely high speeds. That gives you some idea of how densely crowded the 5 billion proteins in a typical cell are, said Anthony Hyman, a British cell biologist and a director of the Max Planck Institute of Molecular Cell Biology and Genetics in Dresden.
Somehow in that bustling cytoplasm, enzymes need to find their substrates, and signaling molecules need to find their receptors, so the cell can carry out the work of growing, dividing and surviving. If cells were sloshing bags of evenly mixed cytoplasm, that would be difficult to achieve. But they are not. Membrane-bounded organelles help to organize some of the contents, usefully compartmentalizing sets of materials and providing surfaces that enable important processes, such as the production of ATP, the biochemical fuel of cells. But, as scientists are still only beginning to appreciate, they are only one source of order.
Recent experiments reveal that some proteins spontaneously gather into transient assemblies called condensates, in response to molecular forces that precisely balance transitions between the formation and dissolution of droplets inside the cell. Condensates, sometimes referred to as membraneless organelles, can sequester specific proteins from the rest of the cytoplasm, preventing unwanted biochemical reactions and greatly increasing the efficiency of useful ones. These discoveries are changing our fundamental understanding of how cells work.
For instance, condensates may explain the speed of many cellular processes. “The key thing about a condensate — it’s not like a factory; it’s more like a flash mob. You turn on the radio, and everyone comes together, and then you turn it off and everyone disappears,” Hyman said.
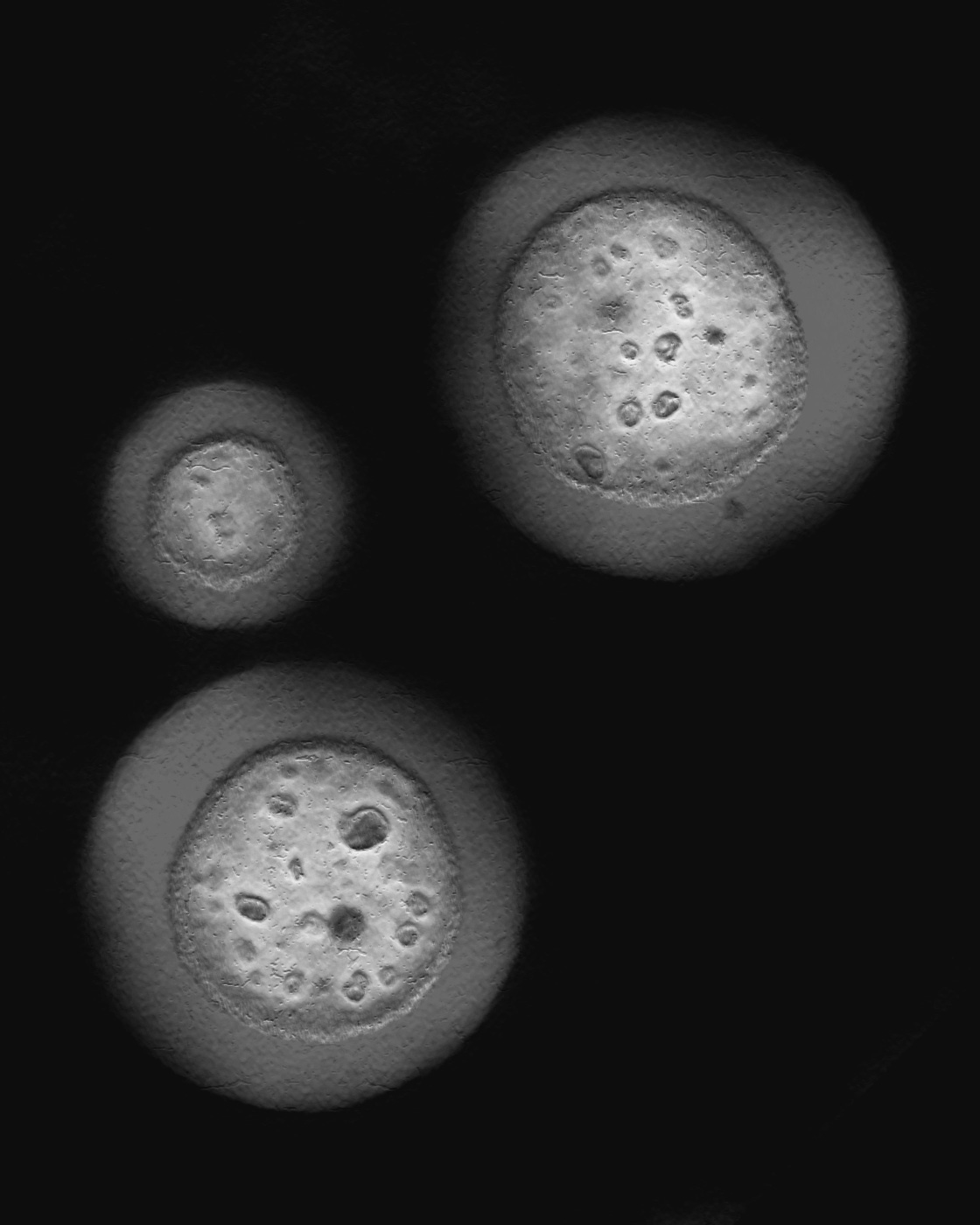
The nucleolus, the largest structure inside the nucleus, is a condensate with internal structure. In these stained nucleoli from frog cells, condensates of different proteins nest within one another.
As such, the mechanism is “exquisitely regulatable,” said Gary Karpen, a cell biologist at the University of California, Berkeley, and the Lawrence Berkeley National Laboratory. “You can form these things and dissolve them quite readily by just changing concentrations of molecules” or chemically modifying the proteins. This precision provides leverage for control over a host of other phenomena, including gene expression.
The first hint of this mechanism arrived in the summer of 2008, when Hyman and his then-postdoctoral fellow Cliff Brangwynne (now a Howard Hughes Medical Institute investigator at Princeton University) were teaching at the famed Marine Biological Laboratory physiology course and studying the embryonic development of C. elegans roundworms. When they and their students observed that aggregates of RNA in the fertilized worm egg formed droplets that could split away or fuse with each other, Hyman and Brangwynne hypothesized that these “P granules” formed through phase separation in the cytoplasm, just like oil droplets in a vinaigrette.
That proposal, published in 2009 in Science, didn’t get much attention at the time. But more papers on phase separation in cells trickled out around 2012, including a key experiment in Michael Rosen’s lab at the University of Texas Southwestern Medical Center in Dallas, which showed that cell signaling proteins can also exhibit this phase separation behavior. By 2015, the stream of papers had turned into a torrent, and since then there’s been a veritable flood of research on biomolecular condensates, these liquid-like cell compartments with both elastic and viscous properties.
Samuel Velasco/Quanta Magazine; Source: Salman F. Banani et al.
Now cell biologists seem to find condensates everywhere they look: in the regulation of gene expression, the formation of mitotic spindles, the assembly of ribosomes, and many more cellular processes in the nucleus and cytoplasm. These condensates aren’t just novel but thought-provoking: The idea that their functions emerge from the collective behaviors of the molecules has become the central concept in condensate biology, and it contrasts sharply with the classic picture of pairs of biochemical agents and their targets fitting together like locks and keys. Researchers are still figuring out how to probe the functionality of these emergent properties; that will require the development of new techniques to measure and manipulate the viscosity and other properties of tiny droplets in a cell.
What Drives Droplet Formation
When biologists were first trying to explain what drives the phase separation phenomenon behind condensation in living cells, the structure of the proteins themselves offered a natural place to start. Well-folded proteins typically have a mix of hydrophilic and hydrophobic amino acids. The hydrophobic amino acids tend to bury themselves inside the protein folds, away from water molecules, while the hydrophilic amino acids get drawn to the surface. These hydrophobic and hydrophilic amino acids determine how the protein folds and holds its shape.
But some protein chains have relatively few hydrophobic amino acids, so they have no reason to fold. Instead, these intrinsically disordered proteins (IDPs) fluctuate in form and engage in many weak multivalent interactions. IDP interactions were thought for years to be the best explanation for the fluidlike droplet behavior.
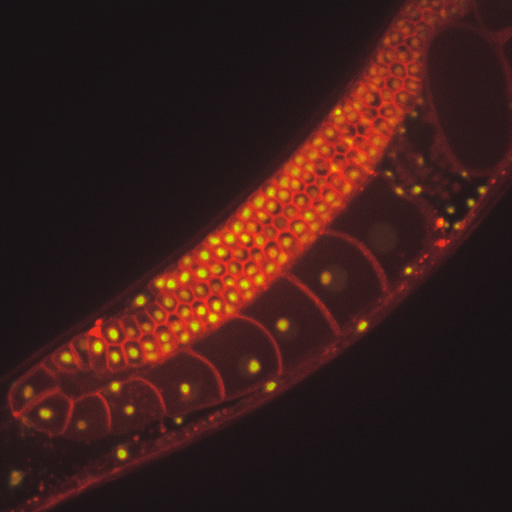
Nucleoli appear as green dots in this stained tissue from a roundworm. Each cell, regardless of its size, has a single nucleolus. Recent research has shown that the size of nucleoli depends on the concentration of nucleolar proteins in a cell.
Stephanie Weber
Last year, however, Brangwynne published a couple of papers highlighting that IDPs are important, but that “the field has gone too far in emphasizing them.” Most proteins involved in condensates, he says, have a common architecture with some structured domains and some disordered regions. To seed condensates, the molecules must have many weak multivalent interactions with others, and there’s another way to achieve that: oligomerization.
Oligomerization occurs when proteins bind to each other and form larger complexes with repeating units, called oligomers. As the concentration of proteins increases, so does the phase separation and the oligomer formation. In a talk at the American Society for Cell Biology meeting in December, Brangwynne showed that as the concentration of oligomers increases, the strength of their interactions eventually overcomes the nucleation barrier, the energy required to create a surface separating the condensate from the rest of the cytoplasm. At that point, the proteins are containing themselves within a droplet.
In the past five years, researchers have taken big strides in understanding how this collective behavior of proteins arises from tiny physical and chemical forces. But they are still learning how (and whether) cells actually use this phenomenon to grow and divide.
Condensates and Gene Expression
Condensates seem to be involved in many aspects of cellular biology, but one area that has received particular attention is gene expression and the production of proteins.
Ribosomes are cells’ protein-making factories, and the number of them in a cell often limits its rate of growth. Work by Brangwynne and others suggests that fast-growing cells might get some help from the biggest condensate in the nucleus: the nucleolus. The nucleolus facilitates the rapid transcription of ribosomal RNAs by gathering up all of the required transcription machinery, including the specific enzyme (RNA polymerase I) that makes them.
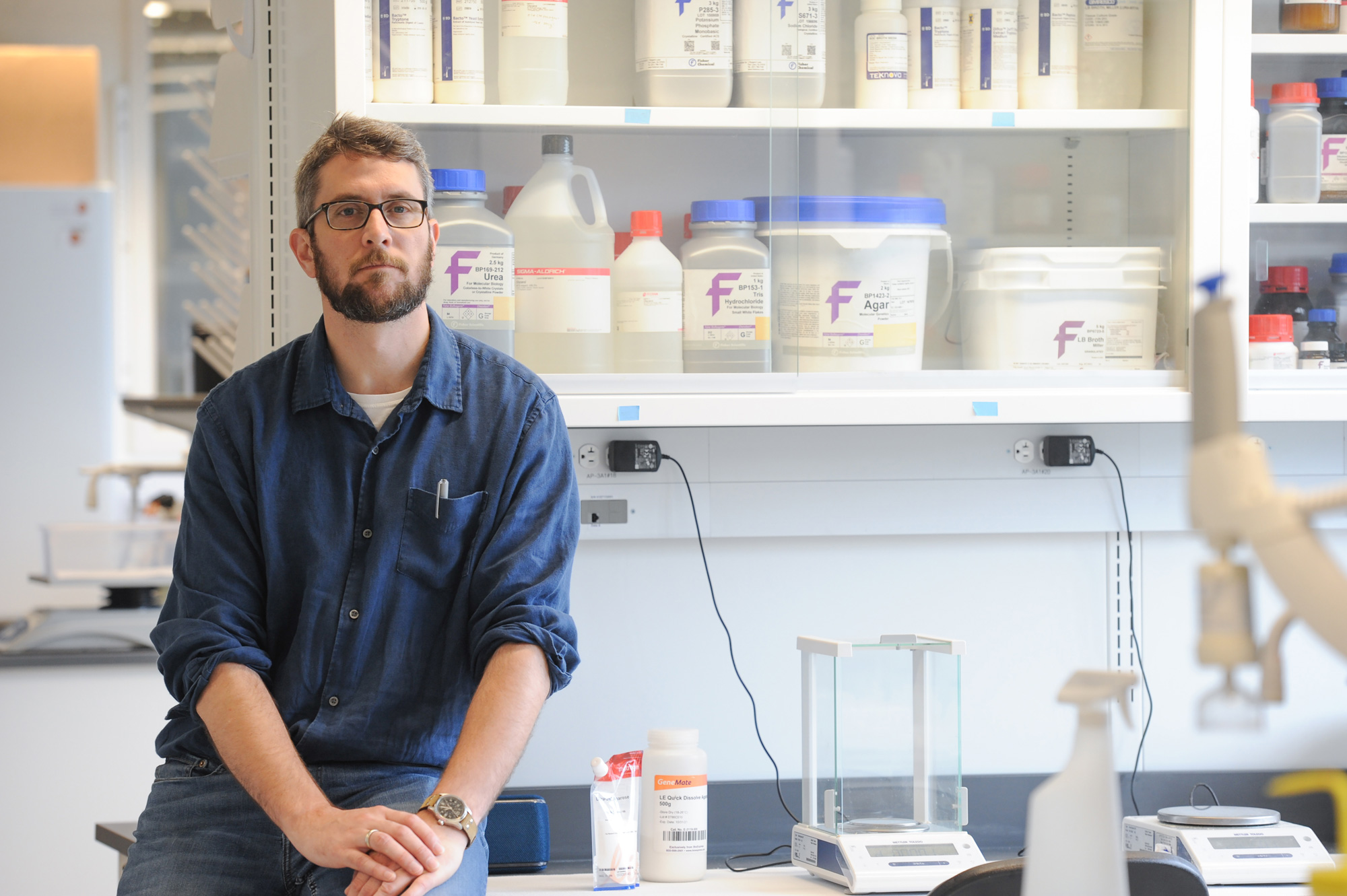
Cliff Brangwynne of Princeon University has argued that the formation of condensates may often be driven by the process of oligomerization, in which proteins bind together as large complexes with repeating subunits.
A few years ago, Brangwynne and his then-postdoc Stephanie Weber, who is now an assistant professor at McGill University in Montreal, investigated how the size of the nucleolus (and therefore the speed of ribosomal RNA synthesis) was controlled in early C. elegans embryos. Because the mother worm contributes the same number of proteins to every embryo, small embryos have high concentrations of proteins and large embryos have low concentrations. And as the researchers reported in a 2015 Current Biology paper, the size of the nucleoli is concentration-dependent: Small cells have large nucleoli and large cells have small ones.
Brangwynne and Weber found that by artificially changing cell size, they could raise and lower the protein concentration and the size of the resulting nucleoli. In fact, if they lowered the concentration below a critical threshold, there was no phase separation and no nucleolus. The researchers derived a mathematical model based on the physics of condensate formation that could exactly predict the size of nucleoli in cells.
Now Weber is looking for condensates in bacteria, which have smaller cells and no membrane-bound compartments. “Maybe this is an even more important mechanism for compartmentalization, because they [bacteria] don’t have an alternative,” she suggested.
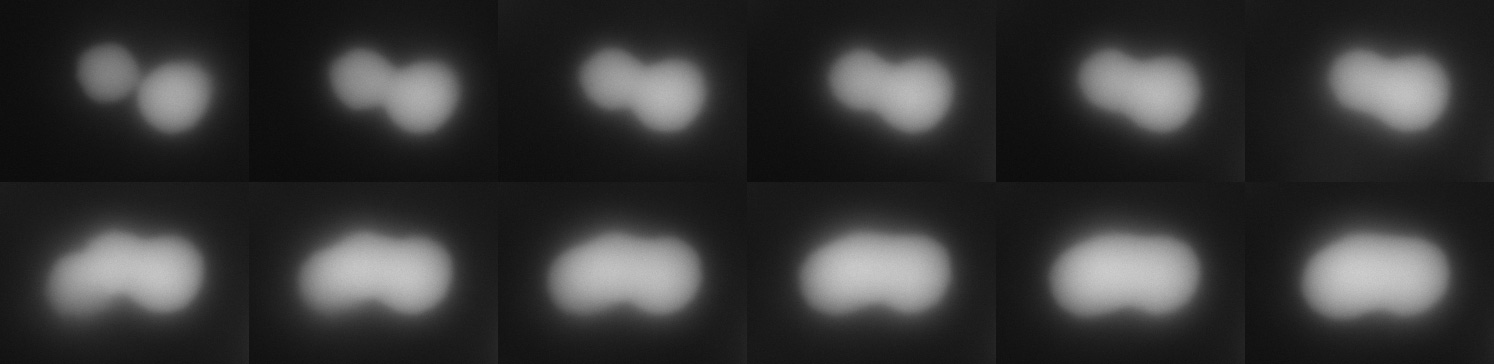

In this series of images, purified bacterial transcription factor in solution acts like a fluid by condensing into spherical droplets that then fuse together. Researchers are studying whether condensates might play a role in regulating bacterial cells as well as eukaryotic ones.
James Wall
Last summer, Weber published a study showing that in cells of slow-growing E. coli bacteria, the RNA polymerase enzyme is uniformly distributed, but in fast-growing cells it clusters in droplets. The fast-growing cells may need to concentrate the polymerase around ribosomal genes to synthesize ribosomal RNA efficiently.
“It looks like it [phase separation] is in all domains of life, and a universal mechanism that has then been able to specialize into a whole bunch of different functions,” Weber said.
Although Weber and Brangwynne showed that active transcription occurs in one large condensate, the nucleolus, other condensates in the nucleus do the opposite. Large portions of the DNA in the nucleus are classified as heterochromatin because they are more compact and generally not expressed as proteins. In 2017, Karpen, Amy Strom (who is now a postdoc in Brangwynne’s lab) and their colleagues showed that a certain protein will undergo phase separation and form droplets on the heterochromatin in Drosophila embryos. These droplets can fuse with each other, possibly providing a mechanism for compacting heterochromatin inside the nucleus.
The results also suggested an exciting possible explanation for a long-standing mystery. Years ago, geneticists discovered that if they took an actively expressed gene and placed it right next to the heterochromatin, the gene would be silenced, as if the heterochromatin state was spreading. “This phenomenon of spreading was something that arose early on, and no one really understood it,” Karpen said.
Later, researchers discovered enzymes involved in epigenetic regulation called methyltransferases, and they hypothesized that the methyltransferases would simply proceed from one histone to the next down the DNA strand from the heterochromatin into the adjacent euchromatin, a kind of “enzymatic, processive mechanism,” Karpen said. This has been the dominant model to explain the spreading phenomenon for the last 20 years. But Karpen thinks that the condensates that sit on the heterochromatin, like wet beads on a string, could be products of a different mechanism that accounts for the spreading of the silent heterochromatin state. “These are fundamentally different ways to think about how the biology works,” he said. He’s now working to test the hypothesis.
The Formation of Filaments
Condensates also helped to solve a different cellular mystery — not inside the nucleus, but along the cell membrane. When a ligand binds to a receptor protein on a cell’s surface, it initiates a cascade of molecular changes and movements that convey a signal through the cytoplasm. But for that to happen, something first has to gather together all the dispersed players in the mechanism. Researchers now think phase separation might be a trick cells use to cluster the required signaling molecules at the membrane receptor, explains Lindsay Case, who trained in the Rosen lab as a postdoc and is starting her own lab at the Massachusetts Institute of Technology this month.
Case notes that protein modifications that are commonly used for transducing signals, such as the addition of phosphoryl groups, change the valency of a protein — that is, its capacity to interact with other molecules. The modifications therefore also affect proteins’ propensity to form condensates. “If you think about what a cell is doing, it is actually regulating this parameter of valency,” Case said.
Condensates may also play an important role in regulating and organizing the polymerization of small monomer subunits into long protein filaments. “Because you’re bringing molecules together for a longer period of time than you would outside the condensate, that favors polymerization,” Case said. In her postdoctoral research, she found that condensates enhance the polymerization of actin into filaments that help specialized kidney cells maintain their unusual shapes.
The polymerization of tubulin is key to the formation of the mitotic spindles that help cells divide. Hyman became interested in understanding the formation of mitotic spindles during his graduate studies in the Laboratory of Molecular Biology at the University of Cambridge in the 1980s. There, he studied how the single-celled C. elegans embryo forms a mitotic spindle before splitting into two cells. Now he’s exploring the role of condensates in this process.
Samuel Velasco/Quanta Magazine; Source: Amy R. Strom et al.
In one in vitro experiment, Hyman and his team created droplets of the microtubule-binding tau protein and then added tubulin, which migrates into the tau droplets. When they added nucleotides to the drops to simulate polymerization, the tubulin monomers assembled into beautiful microtubules. Hyman and his colleagues have proposed that phase separation could be a general way for cells to initiate the polymerization of microtubules and the formation of the mitotic spindle.
The tau protein is also known for forming the protein aggregates that are the hallmarks of Alzheimer’s disease. In fact, many neurodegenerative conditions, such as amyotrophic lateral sclerosis (ALS) and Parkinson’s disease, involve the faulty formation of protein aggregates in cells.
To investigate how these aggregates might form, Hyman’s team focused on a protein called FUS that has mutant forms associated with ALS. The FUS protein is normally found in the nucleus, but in stressed cells, the protein leaves the nucleus and goes into the cytoplasm, where it forms into droplets. Hyman’s team found that when they made droplets of mutated FUS proteins in vitro, after only about eight hours the droplets solidified into what he calls “horrible aggregates.” The mutant proteins drove a liquid-to-solid phase transition far faster than normal form of FUS did.
Maybe the question isn’t why the aggregates form in disease, but why they don’t form in healthy cells. “One of the things I often ask in group meetings is: Why is the cell not scrambled eggs?” Hyman said in his talk at the cell biology meeting; the protein content of the cytoplasm is “so concentrated that it should just crash out of solution.”
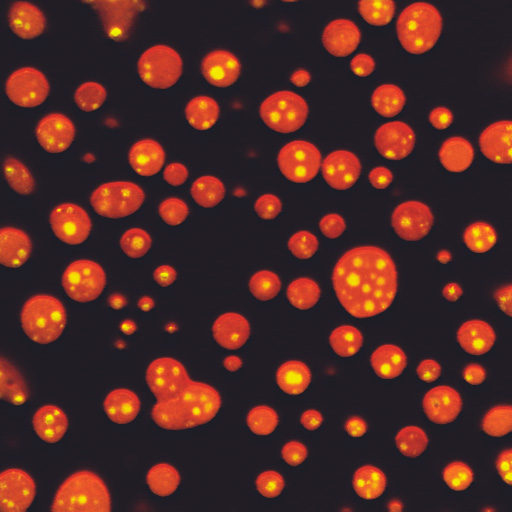
Two types of proteins (red, yellow) isolated from the nucleoli of frog eggs can spontaneously organize into condensate droplets. By altering the concentrations of each protein in the solution, researchers can make either or both of the types of condensates grow or disappear.
A clue came when researchers in Hyman’s lab added the cellular fuel ATP to condensates of purified stress granule proteins and saw those condensates vanish. To investigate further, the researchers put egg whites in test tubes, added ATP to one tube and salt to the other, and then heated them. While the egg whites in the salt aggregated, the ones with ATP did not: The ATP was preventing protein aggregation at the concentrations found in living cells.
But how? It remained a puzzle until Hyman fortuitously met a chemist when presenting a seminar in Bangalore. The chemist noted that in industrial processes, additives called hydrotropes are used to increase the solubility of hydrophobic molecules. Returning to his lab, Hyman and his colleagues found that ATP worked exceptionally well as a hydrotrope.
Intriguingly, ATP is a very abundant metabolite in cells, with a typical concentration of 3-5 millimolar. Most enzymes that use ATP operate efficiently with concentrations three orders of magnitude lower. Why, then, is ATP so concentrated inside cells, if it isn’t needed to drive metabolic reactions?
One candidate explanation, Hyman suggests, is that ATP doesn’t act as a hydrotrope below 3-5 millimolar. “One possibility is that in the origin of life, ATP might have evolved as a biological hydrotrope to keep biomolecules soluble in high concentration and was later co-opted as energy,” he said.
It’s difficult to test that hypothesis experimentally, Hyman admits, because it is challenging to manipulate ATP’s hydrotropic properties without also affecting its energy function. But if the idea is correct, it might help to explain why protein aggregates commonly form in diseases associated with aging, because ATP production becomes less efficient with age.
Other Uses for Droplets
Protein aggregates are clearly bad in neurodegenerative diseases. But the transition from liquid to solid phases can be adaptive in other circumstances.
Take primordial oocytes, cells in the ovaries that can lie dormant for decades before maturing into an egg. Each of these cells has a Balbiani body, a large condensate of amyloid protein found in the oocytes of organisms ranging from spiders to humans. The Balbiani body is believed to protect mitochondria during the oocyte’s dormant phase by clustering a majority of the mitochondria together with long amyloid protein fibers. When the oocyte starts to mature into an egg, those amyloid fibers dissolve and the Balbiani body disappears, explains Elvan Böke, a cell and developmental biologist at the Center for Genomic Regulation in Barcelona. Böke is working to understand how these amyloid fibers assemble and dissolve, which could lead to new strategies for treating infertility or neurodegenerative diseases.
Protein aggregates can also solve problems that require very quick physiological responses, like stopping bleeding after injury. For example, Mucor circinelloides is a fungal species with interconnected, pressurized networks of rootlike hyphae through which nutrients flow. Researchers at the Temasek Life Sciences Laboratory led by the evolutionary cell biologist Greg Jedd recently discovered that when they injured the tip of a Mucor hypha, the protoplasm gushed out at first but almost instantaneously formed a gelatinous plug that stopped the bleeding.
Jedd suspected that this response was mediated by a long polymer, probably a protein with a repetitive structure. The researchers identified two candidate proteins and found that, without them, injured fungi catastrophically bled out into a puddle of protoplasm.
Jedd and his colleagues studied the structure of the two proteins, which they called gellin A and gellin B. The proteins had 10 repetitive domains, some of which had hydrophobic amino acids that could bind to cell membranes. The proteins also unfolded at forces similar to those they would experience when the protoplasm comes gushing out at the site of an injury. “There’s this massive acceleration in flow, and so we were thinking that maybe this is the trigger that is telling the gellin to change its state,” Jedd said. The plug, triggered by a physical cue that causes the gellin to transition from liquid to solid phase, is irreversibly solidified.
In contrast, in the fungal species Neurospora, the hyphae are divided into compartments, with pores that regulate the flow of water and nutrients. Jedd wanted to know how the pores were opened and closed. “What we discovered is some intrinsically disordered proteins that seem to be undergoing a condensation to aggregate at the pore, to provide a mechanism for closing it,” Jedd explained.
The Neurospora proteins that were candidates for this job, Jedd’s team learned, had repeated mixed-charge domains that could be found in some mammalian proteins, too. When the researchers synthesized proteins of varying compositions but with similar mixes of lengths and charge patterning and introduced them into mammalian cells, they found that the proteins could be incorporated into nuclear speckles, which are condensates in the mammalian cell nucleus that help to regulate gene expression, as they and colleagues led by Rohit Pappu of Washington University in St. Louis reported in a 2020 Molecular Cell paper.
The fungal and mammalian kingdoms seem to have arrived independently at a strategy of using disordered sequences in mechanisms based on condensation, Jedd said, “but they’re using it for entirely different reasons, in different compartments.”
Reconsidering Old Explanations
Phase separation has turned out to be ubiquitous, and researchers have generated lots of ideas about how this phenomenon could be involved in various cell functions. “There’s lots of exciting possibilities that [phase separation] raises, so that’s what I think drives … interest in the field,” Karpen said. But he also cautions that while it is relatively easy to show that a molecule undergoes phase separation in a test tube, demonstrating that phase separation has a function in the cell is much more challenging. “We still don’t know so much,” he said.
Brangwynne agreed. “If you’re really honest, it’s still pretty much at a hand-wavy stage, the whole field,” he said. “It’s very early days for understanding how this all works. The fact that it’s hand-wavy doesn’t mean that liquid phase separation isn’t the key driving force. In fact, I think it is. But how does it really work?”
The uncertainties do not discourage Hyman, either. “What phase separation is allowing everyone to do is go back and look at old problems which stalled out and think: Can we now think about this a different way?” he said. “All the structural biology that was done has just been brilliant — but many problems stalled out. They couldn’t actually explain things. And that’s what phase separation has allowed, is for everyone to think again about these problems.”
This article was reprinted on Wired.com.