What Makes Life Tick? Mitochondria May Keep Time for Cells
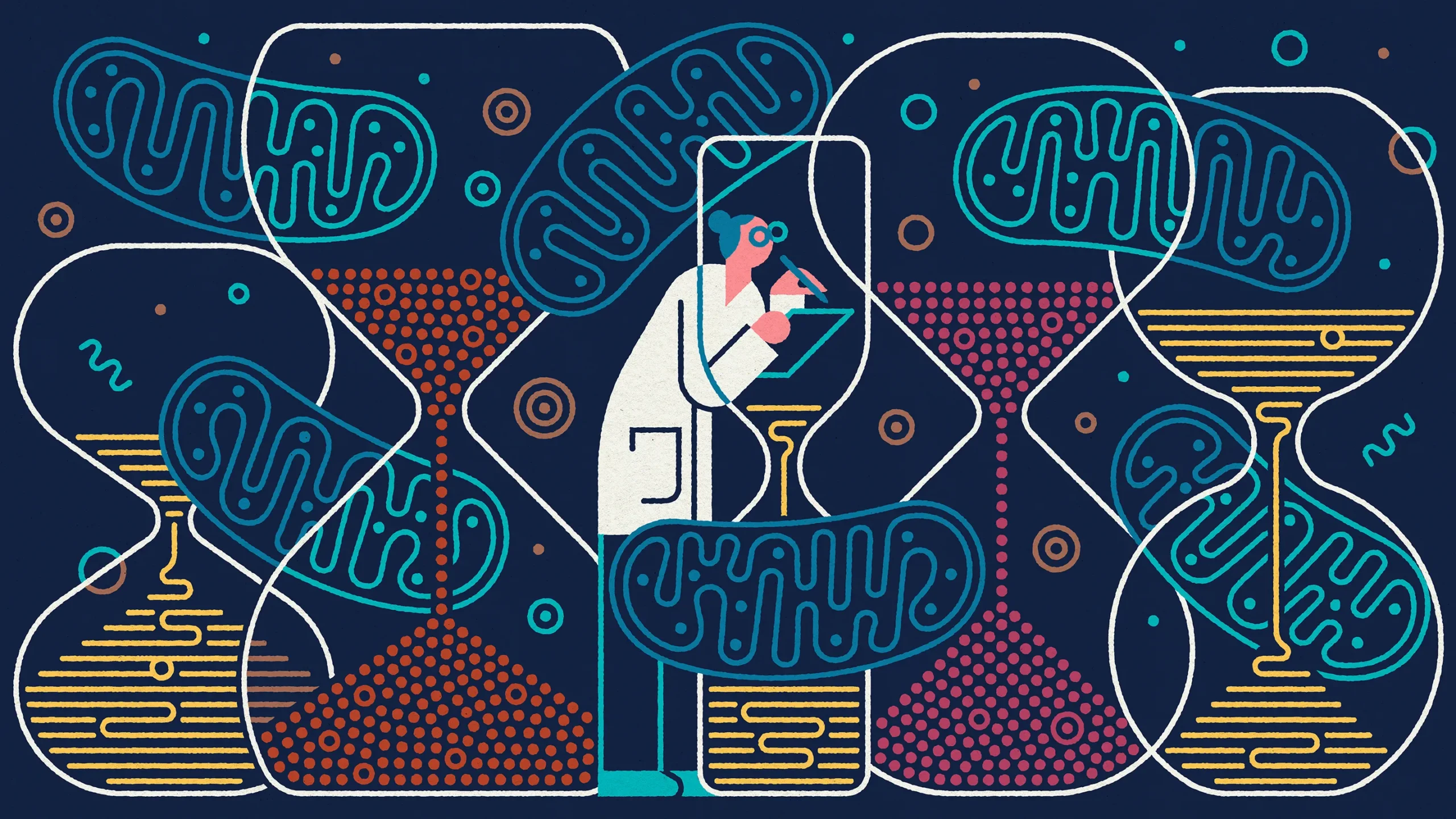
Carlos Arrojo for Quanta Magazine
Introduction
Just as people in different places seem to operate at different rhythms, so too do different species. They age at their own rates: Some, like the fruit fly, race to adulthood so they can reproduce before their ephemeral food source disappears, while creatures like humans mature slowly over decades, in part because building a large, complex brain requires it. And at the very beginning of an embryo’s life, small tweaks in the timing of when and how different tissues develop can dramatically alter an organism’s form — a mechanism that evolution exploits in creating new species. However, what sets the tempo of an organism’s growth has remained a mystery.
“Our knowledge of what controls developmental timing has really lagged behind other areas in developmental biology,” said Margarete Diaz Cuadros, who leads research focused on developmental tempo at Massachusetts General Hospital in Boston.
Developmental biologists have had tremendous success in identifying networks of regulatory genes that talk to one another — cascading systems of feedback loops that turn genes on or off at exactly the right time and place to build, say, an eye or a leg. But the highly conserved similarity in these gene networks among species contrasts with huge differences in developmental timing. Mice and humans, for example, use the same sets of genes to create neurons and build spines. Yet the brain and spine of a mouse turn out quite differently than those of a human because the timing of when those genes are active is different, and it’s unclear why that’s so.
“Gene regulation does not seem to explain everything about developmental timing,” said Pierre Vanderhaeghen, who studies the evolution and development of the brain at KU Leuven in Belgium. “Now, this is a bit provocative because in a way, in biology, everything should be explained by gene regulation, directly or indirectly.”
New explanations for what makes life tick are emerging from innovations — like advances in stem cell culture and the availability of tools to manipulate metabolism, initially developed to study cancer — that now allow researchers to chart, and toy with, the pace of development of early embryos and tissues in greater detail. In a string of papers over the past few years, including one key publication in June, several research teams have independently converged on intriguing connections between the tempo of development, the pace of biochemical reactions, and rates of gene expression underlying those biochemical reactions.
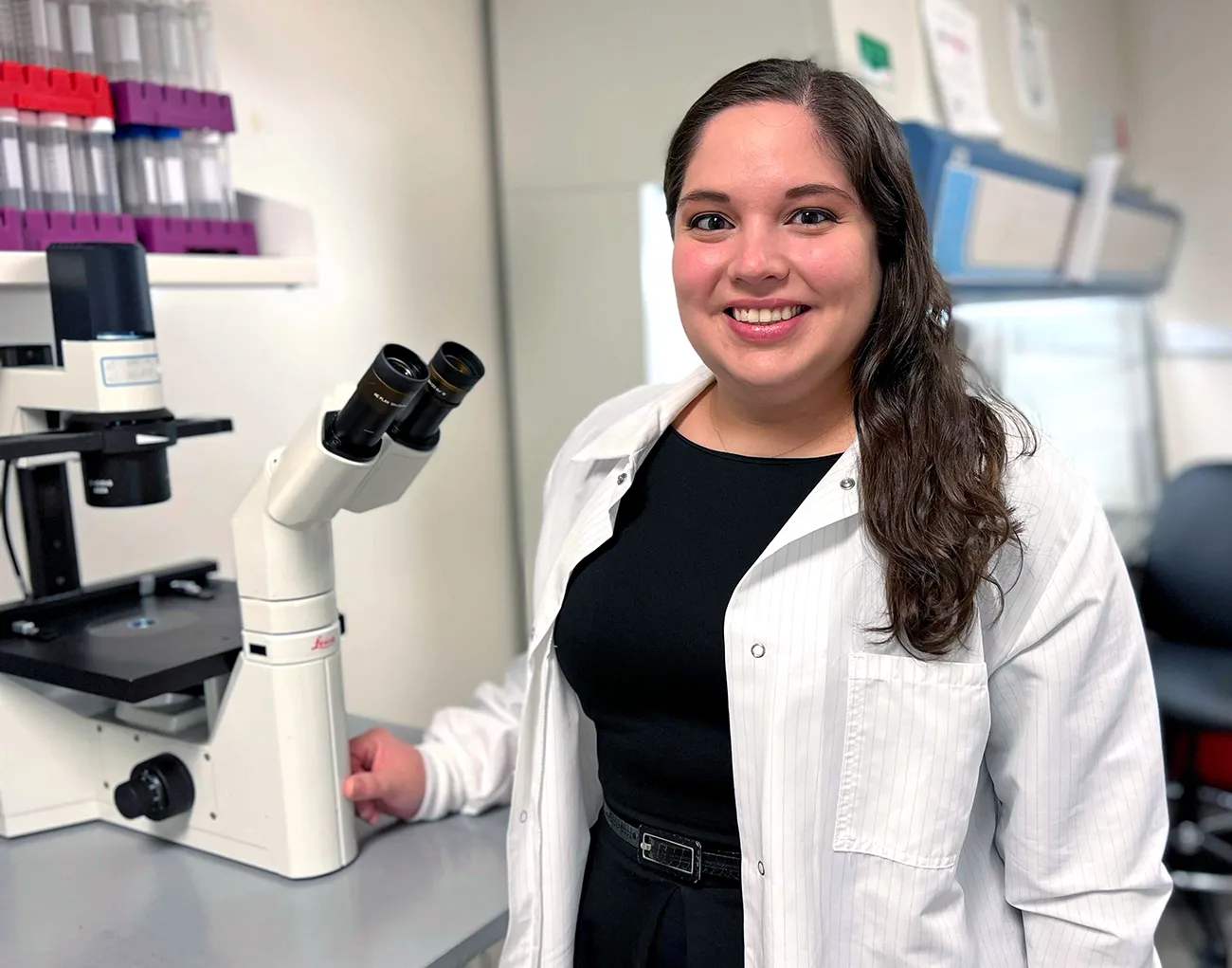
Margarete Diaz Cuadros and her colleagues at Harvard Medical School created a model of the segmentation clock, which patterns the vertebrate spine, in mouse and human stem cells.
Alejandra Rodriguez de la Rosa
Their findings point to a common metronome: the mitochondria, which may be the timekeeper of the cell, setting the rhythm for a variety of developmental and biochemical processes that create and maintain life.
A Neuron Keeps Time
More than a decade ago, Vanderhaeghen did an experiment that laid the foundation for modern studies about how developmental tempo is kept. The neurobiologist was in his Belgian lab growing stem cells in petri dishes and observing how long they took to mature from cellular blank slates to full-fledged neurons connecting and communicating with others. He thought he might find clues to the origin and evolution of the human brain by comparing these mouse and human stem cells primed to become neurons.
The first thing he noticed was that mouse stem cells differentiated into mature brain cells in about a week — more quickly than human stem cells, which took their time growing over three to four months.
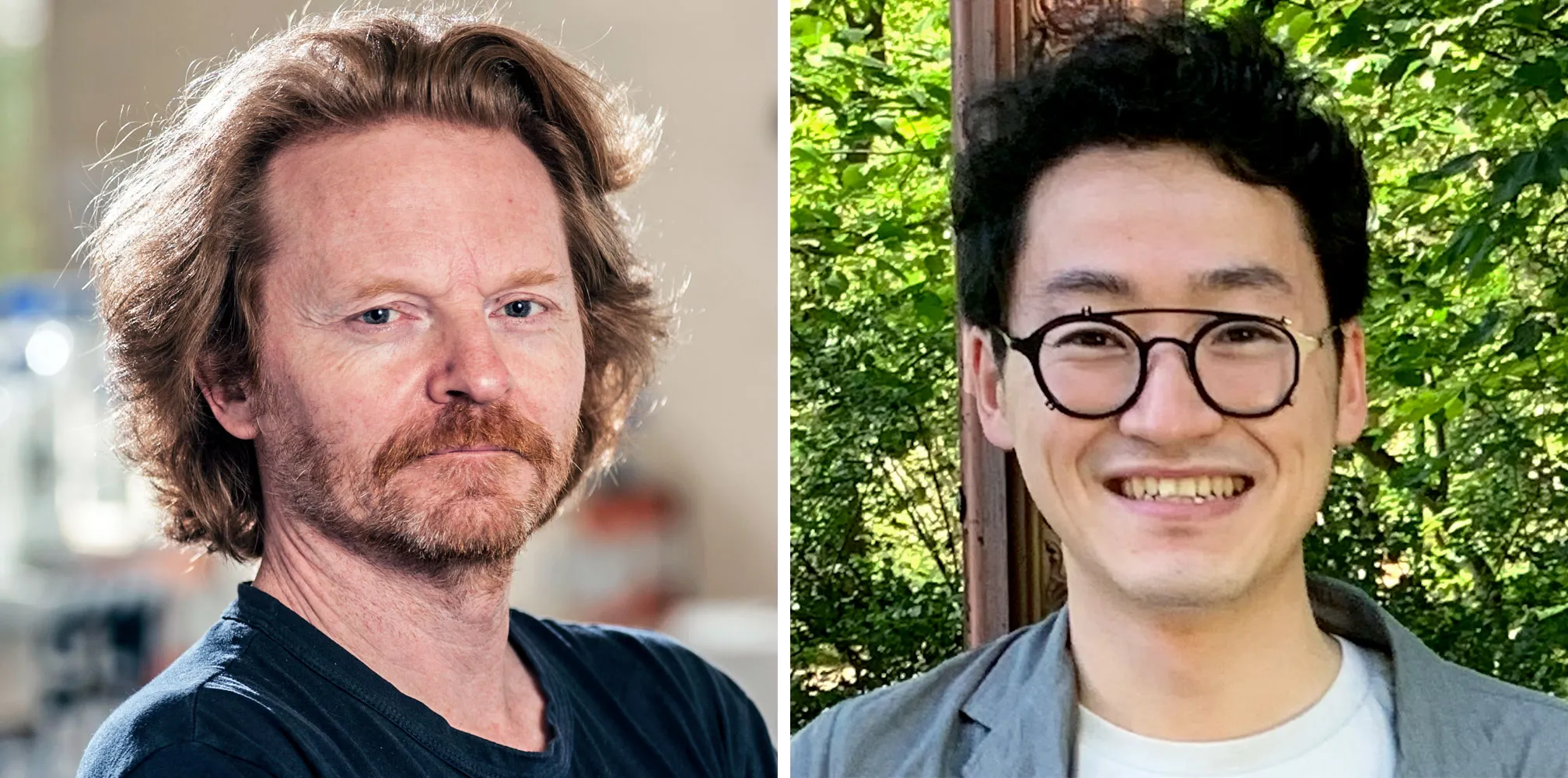
More than a decade ago, the neurobiologist Pierre Vanderhaeghen (left) at KU Leuven in Belgium discovered that the tempo of development is intrinsic to the cell itself. More recently, he and Ryohei Iwata (right) have studied whether mitochondria may be the driving force behind it.
Courtesy of Pierre Vanderhaeghen (left); Courtesy of Ryohei Iwata
But would those cells develop the same way in a growing brain rather than in an isolated dish? To find out, he transplanted a mouse neuron into a live mouse brain. The cell followed the same timeline as the neurons of the host mouse, differentiating after about one week. Then he tried the same thing with a human neuron, implanting it into a mouse brain. To his amazement, the human neuron kept its own time. It took nearly a year to mature despite its rodential environment.
“That provided us a first important answer, which is that whatever the timing mechanism is, a lot of it seems to be in the neurons themselves,” Vanderhaeghen said. “Even if you take the cells out of the petri dish and put them in another organism, they will still keep their own timeline.”
Still, virtually nothing was known about the underlying cellular mechanism until a couple of years ago.
Vanderhaeghen started thinking about where the building blocks of a neuron come from. “To make neurons, it’s like building a super complicated building,” he said. “You need some good logistics.” Cells need not only energy but a source of raw materials to grow and divide.
He suspected that mitochondria might provision these building blocks. The organelles are key to a cell’s growth and metabolism. They produce energy, earning them the nickname “the powerhouse of the cell,” and they also produce metabolites essential for constructing amino acids and nucleotides and for regulating gene expression.
The classic view of mitochondria is that they don’t change over a cell’s life span. “They’re just this nice, picturesque little sausage in the cell, and they provide energy,” Vanderhaeghen said. But when he and Ryohei Iwata, a postdoctoral scholar in his lab, looked more closely at developing neurons, they saw that mitochondria need time to develop as well.
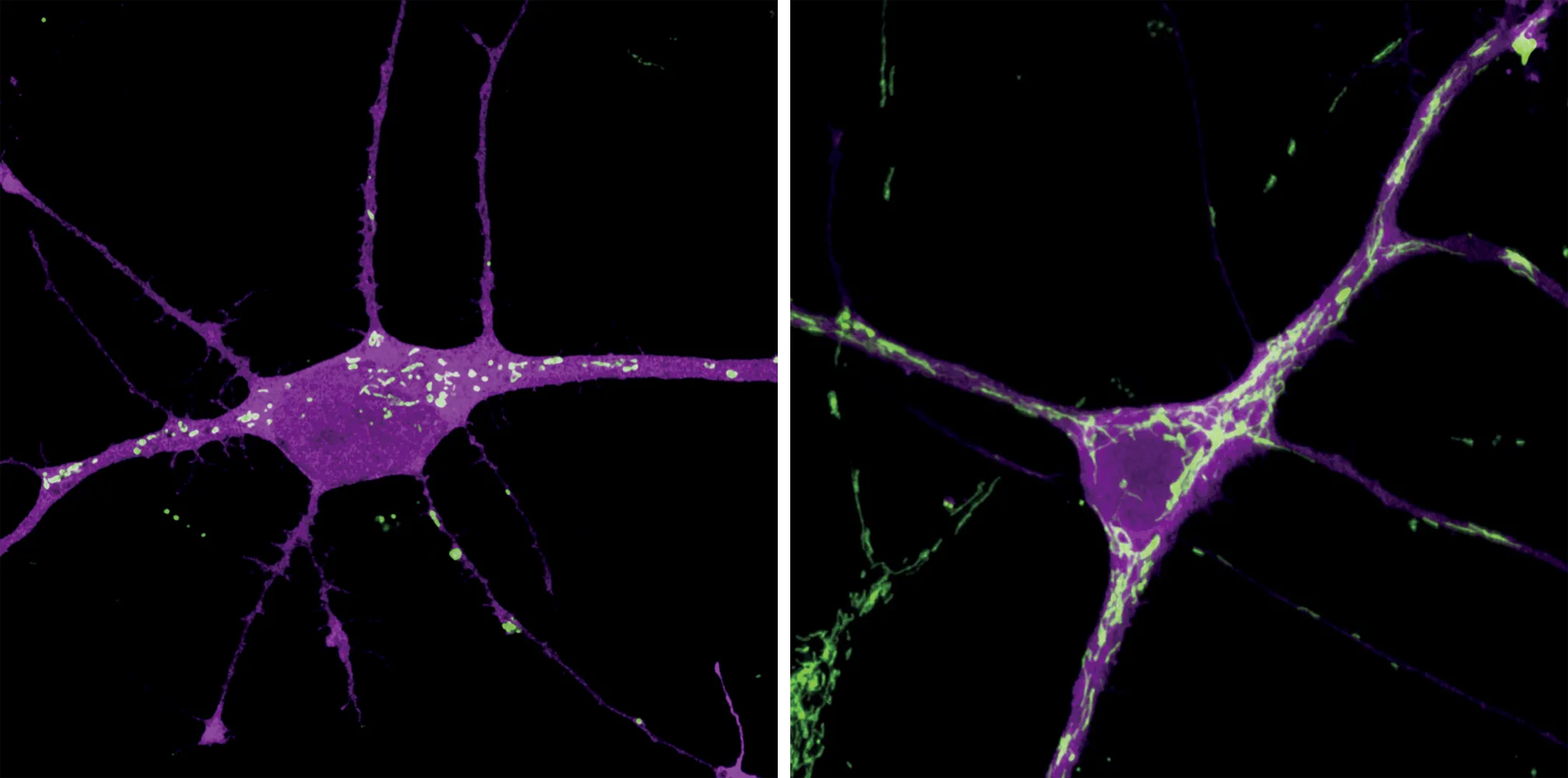
Mitochondria (stained green) are not static over a cell’s life span. As a young neuron (left) matures, the organelles grow in number, generate more energy, and take on their characteristic bean shape (right).
Ryohei Iwata
Young neurons, they reported in Science, had few mitochondria, and the ones they had were fragmented and generated little energy. Then, as the neurons matured, the mitochondria grew in number, size and metabolic activity. What’s more, the changes occurred faster in mice than in humans. Essentially, the system scaled: The maturation of mitochondria stayed in sync with the maturation of neurons in both species.
The discovery struck Vanderhaeghen and Iwata as important. And it made them wonder if mitochondria could be the quiet drumbeat driving the vast differences in developmental tempo among species.
How to Grow a Spine
One of the classic models for studying the tempo of embryonic development is the patterning of the spine. All vertebrates have a spine composed of a string of vertebral segments, but species vary in their number and size. A natural question therefore arises about the developmental mechanisms that give rise to this essential vertebrate feature and its many variations throughout the animal kingdom.
In 1997, the developmental biologist Olivier Pourquié, now at Harvard Medical School, first uncovered a molecular oscillator called a segmentation clock that drives the mechanism that patterns the vertebrate spine. Working with chicken embryos, his research team identified the key players that are rhythmically expressed during the formation of each vertebral segment in embryonic tissue. The segmentation clock triggers oscillations of gene expression, causing cells to fluctuate in their responsiveness to a wavefront signal that moves from head to tail. When the wavefront encounters responsive cells, a segment forms. In this way, the clock-and-wavefront mechanism controls the periodic organization of the spine.
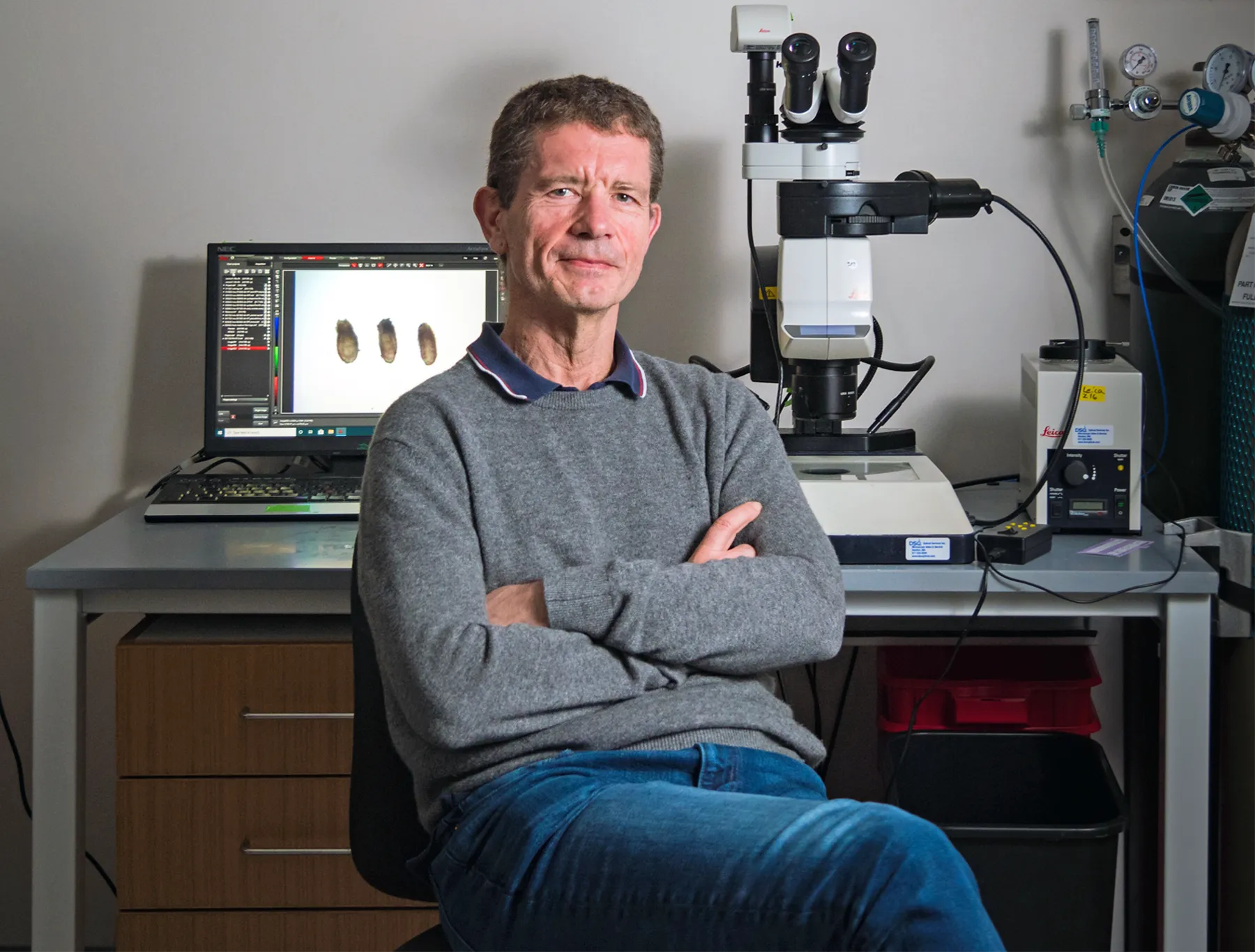
To Olivier Pourquié of Harvard Medical School, research suggests that there is “a global command” that manages all the developmental timing processes.
Amanda Wild
The genes that orchestrate the segmentation clock are conserved across species. However, the clock period — the time between two peaks in an oscillation — is not. For many years, developmental geneticists were at a loss to explain this: They didn’t have the genetic tools to manipulate the clock precisely in a growing embryo. So, around 2008, Pourquié started to develop methods to better dissect the mechanism in the lab.
At that time, “it sounded like total science fiction,” he said. But the idea became more plausible over the following decade, as Pourquié’s lab and others around the world learned to culture embryonic stem cells and even build organoids — like a retina, gut or mini-brain — in a dish.
Pourquié and Diaz Cuadros, then his graduate student, found a way to reproduce the clock in mouse and human stem cells. In early experiments, they observed that the clock period runs about two hours in mice, whereas it takes about five hours to complete an oscillation in human cells. It was the first time anyone had identified the segmentation clock period in humans.
Merrill Sherman/Quanta Magazine
Other labs also saw the potential of these advances in stem cell biology to tackle long-standing questions about developmental timing. In 2020, two research groups — one led by Miki Ebisuya at the European Molecular Biology Laboratory in Barcelona and the other by James Briscoe at the Francis Crick Institute in London — independently discovered that basic molecular processes in the cell stay on beat with the pace of development. They published studies side by side in Science.
Ebisuya’s team wanted to understand differences in the rate of molecular reactions — gene expression and protein degradation — that drive each clock cycle. They found that both processes worked twice as fast in mouse cells as in human ones.
Briscoe looked instead at the early development of the spinal cord. Like the segmentation clock cycle, the neuron differentiation process — including the expression of gene sequences and the breakdown of proteins — was proportionally stretched out in humans compared to mice. “It takes two to three times longer to get to the same stage of development using human embryonic stem cells,” Briscoe said.
It was as if, inside of each cell, a metronome was ticking away. With each swing of the pendulum, a variety of cellular processes — gene expression, protein degradation, cell differentiation and embryonic development — all kept pace and stayed on time.
But was this a general rule for all vertebrates, beyond mice and humans? To find out, Ebisuya’s graduate student Jorge Lázaro created a “stem cell zoo,” home to cells from a variety of mammals: mice, rabbits, cattle, rhinoceroses, humans and marmosets. When he reproduced the segmentation clock of each species, he saw that the speed of biochemical reactions stayed in rhythm with the segmentation clock period in every one.
What’s more, the clock tempos did not scale with the animals’ size. Mouse cells oscillated more quickly than rhinoceros cells, but human cells oscillated more slowly than the rhino cells, and marmoset cells had the slowest oscillations of all.
The findings, published in Cell Stem Cell in June, suggested that the speed of biochemical reactions could be a universal mechanism for regulating developmental time.
They also pushed the bounds of an important but overlooked aspect of the central dogma of molecular biology. “We’re talking about transcription, translation and protein stability,” Diaz-Cuadros said. Everyone had thought that they were the same in all mammalian or vertebrate species, “but now what we’re saying is that the speed of the central dogma is species-specific, and I think that is quite fascinating.”
Make or Break a Protein
The clock, then, must stem from a mechanism that sets the pace of biochemical reactions across species. Teresa Rayon wanted to uncover its origins when she watched motor neurons differentiate in her London laboratory, where she studied under Briscoe.
She genetically engineered developing mouse and human neurons to express fluorescent protein, which glows brightly when excited by a laser at the right wavelength. Then she watched the introduced proteins as they degraded. To her surprise, the very same fluorescent proteins came apart more quickly in mouse cells than in human cells, keeping time with the neurons’ development. That suggested to her that something in the intracellular environment set the tempo of degradation.
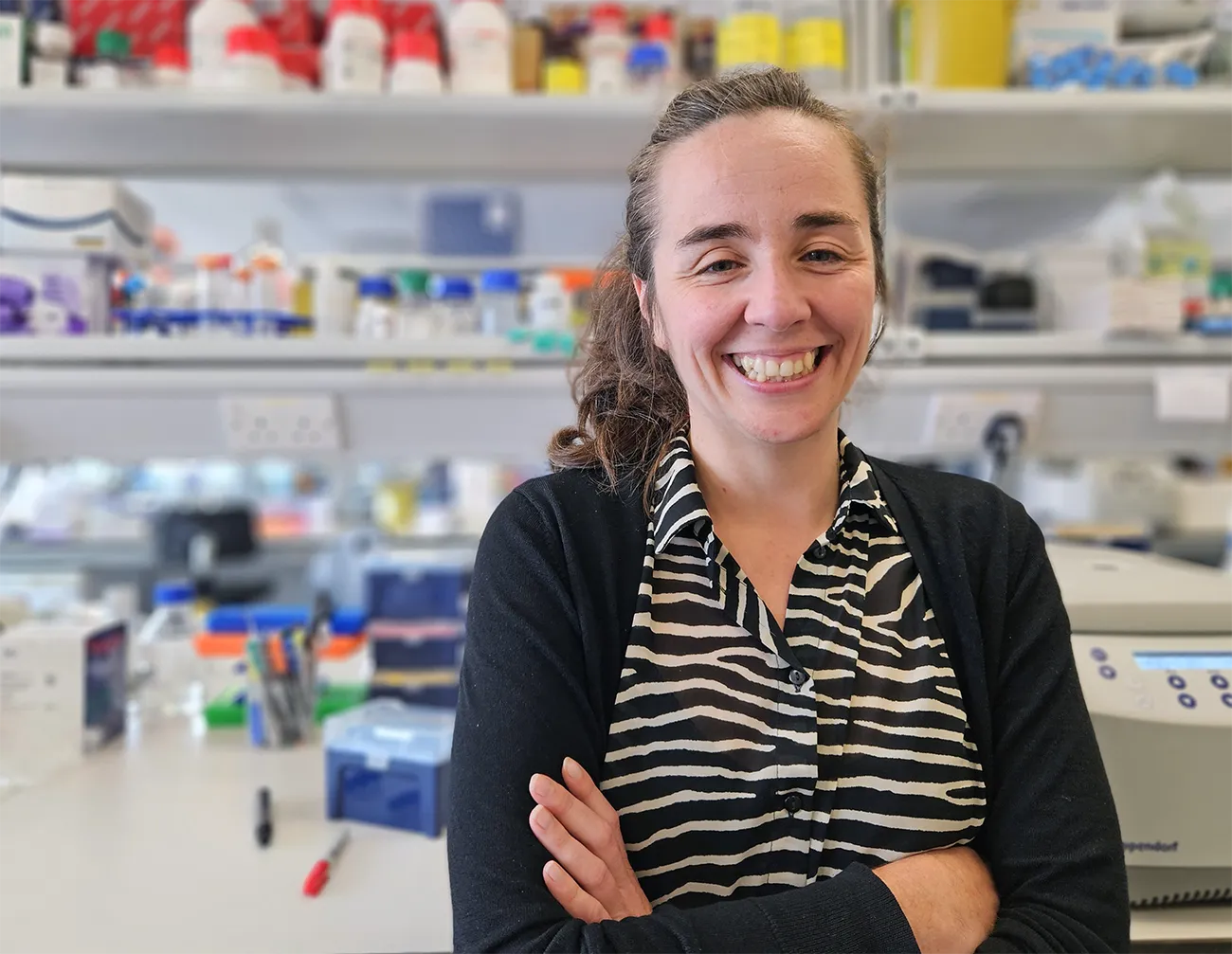
After observing proteins degrade at a consistent rate in developing neurons, Teresa Rayon, now at the Babraham Institute in England, looked for the mechanism that sets the metabolic tempo.
The Babraham Institute
“If you were to ask a biologist, ‘How do you determine the stability of a protein?’ they would tell you that it’s down to the sequence,” said Rayon, who now leads her own lab at the Babraham Institute in Cambridge, England. “However, we found that that’s actually not the case. We think that it might be the machinery that is degrading the proteins that might be playing a role.”
But she and her group were looking in only a single cell type. If cell types in various tissues develop at different rates, would their proteins degrade at different rates, too?
Michael Dorrity at the European Molecular Biology Laboratory in Heidelberg was digging into that question by thinking about how temperature affects development. Many animals, from insects to fish, develop faster when reared at higher temperatures. Intriguingly, he observed that in zebra fish embryos raised in a warm environment, the developmental tempo of some cell types accelerated faster than that of others.
In a preprint he posted last year, he homed in on an explanation involving the machinery that makes and degrades proteins. Some cell types require a greater volume or more complex proteins than others. As a result, some cell types are chronically “putting a load on these protein quality control mechanisms,” he said. When the temperature rises, they don’t have the capacity to keep up with the higher protein needs, and so their internal clock fails to speed up and keep pace.
In that sense, organisms don’t maintain a single unified clock, but have many clocks for many tissues and cell types. Evolutionarily speaking, this is not a bug but a feature: When tissues develop out of sync with one another, body parts can grow at different rates — which can lead to the evolution of diverse organisms or even new species.
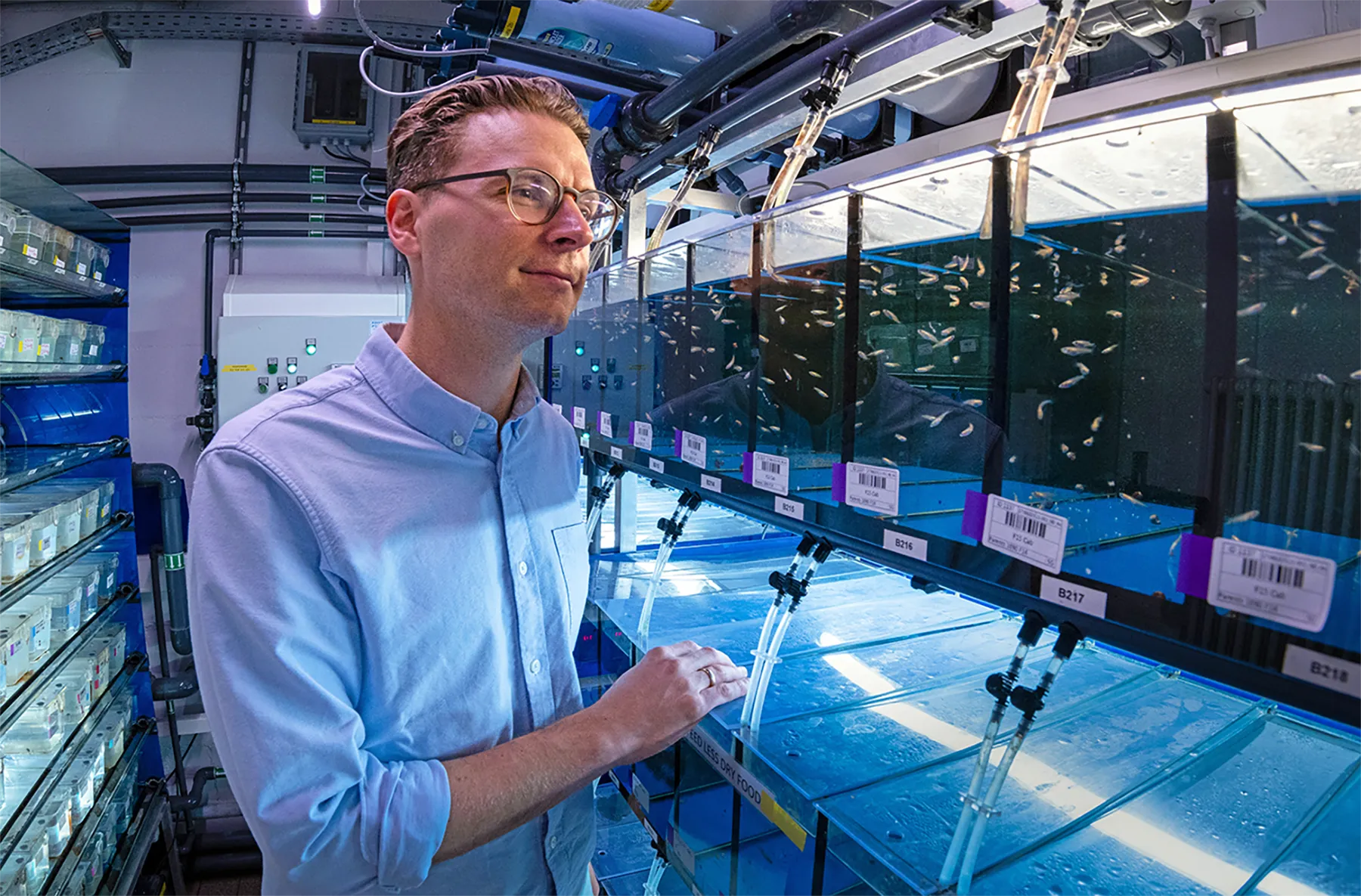
Michael Dorrity at the European Molecular Biology Laboratory in Heidelberg found that every tissue in zebra fish embryos has its own developmental timepiece that determines how quickly the cell type matures.
EMBL Stuart Ingham
So far, these mechanisms across systems and scales — in the developing embryo’s segmentation clock, in a single developing neuron, and in more fundamental protein machinery — have all continued to beat in time.
“Pretty much everything we looked at so far is scaling,” Pourquié said, “which means that there is a global command for all these processes.”
The Tick-Tock of Metabolism
What could this upstream control system be? Pourquié and Diaz Cuadros pondered which system could potentially affect a variety of cellular processes — and they landed on metabolism, driven by mitochondria. Mitochondria produce ATP, the energy currency of the cell, as well as a host of metabolites essential for building proteins and DNA, regulating the genome, and performing other critical processes.
To test that idea, they devised genetic and pharmacological methods to speed up and then slow down the metabolic rates of their stem cells. If mitochondria were indeed setting the cellular tempo, they expected to see their experiments alter the rhythm of the segmentation clock.
When they slowed metabolism in human cells, the segmentation clock slowed too: Its period stretched from five to seven hours, and the rate of protein synthesis slowed as well. And when they sped metabolism up, the clock’s oscillations accelerated, too.
It was as if they had discovered the tuning knob of the cell’s internal metronome, which let them accelerate or decelerate the tempo of embryonic development. “It’s not differences in the gene regulatory architecture that explains these differences in timing,” Pourquié said. The findings were published in Nature earlier this year.
This metabolic tuning knob wasn’t limited to the developing embryo. Iwata and Vanderhaeghen, meanwhile, figured out how to use drugs and genetics to toy with the metabolic tempo of maturing neurons — a process that, unlike that of the segmentation clock, which runs for only a couple of days, takes many weeks or months. When mouse neurons were compelled to generate energy more slowly, the neurons matured more slowly, too. Conversely, by pharmacologically shifting human neurons toward a faster pathway, the researchers could accelerate their maturation. The findings were published in Science in January.
To Vanderhaeghen, the conclusion of their experiments is clear: “Metabolic rate is driving developmental timing.”
Yet, even if metabolism is the upstream regulator of all other cellular processes, those differences must come back to genetic regulation. It’s possible that mitochondria influence the timing of the expression of developmental genes or those involved in the machinery for making, maintaining and recycling proteins.
One possibility, Vanderhaeghen speculated, is that metabolites from the mitochondria are essential to the process that condenses or expands folded DNA in genomes so that it can be transcribed to build proteins. Maybe, he suggested, those metabolites limit the rate of transcription and globally set the pace at which gene regulatory networks are turned on and off. That’s just one idea, however, that needs experimental unpacking.
There is also the question of what makes mitochondria tick in the first place. Diaz Cuadros thinks that the answer must lie in DNA: “Somewhere in their genome, there has to be a sequence difference between mouse and human that is encoding that difference in developmental rate.”
“We still have no idea where that difference is,” she said. “We’re unfortunately still very far from that.”
Finding that answer may take time, and like the mitochondrial clock, scientific progress proceeds at a tempo all its own.
Correction: September 18, 2023
In the introduction, a sentence was revised to clarify that it is the rate of gene expression, not overall metabolic rate, that helps to direct the tempo of development. The article was also updated to correct which species in the stem cell zoo have the fastest and slowest segmentation-clock oscillations.